Contents
- Vitamin K
- Are all forms of vitamin K the same?
- Vitamin K2
- What does Vitamin K do?
- Are you getting enough vitamin K?
- Vitamin K Health Benefits
- How much vitamin K do you need?
- What foods provide vitamin K?
- Vitamin K Supplements
- Vitamin K Side Effects and Toxicity
- Vitamin K Deficiency
- Vitamin K Deficiency causes
- Groups at risk of vitamin K deficiency
- Vitamin K deficiency signs and symptoms
- Vitamin K deficiency complications
- Vitamin K deficiency diagnosis
- Vitamin K deficiency treatment
- Phytonadione (vitamin K1)
- Prophylaxis in newborns
- Vitamin K deficiency bleeding (VKDB) in newborns
- Hereditary combined vitamin K-dependent clotting factor deficiency (VKCFD)
- Vitamin K deficiency due to malabsorption
- Vitamin K deficiency in adults due to poor nutrition
- Vitamin K deficiency due to chronic conditions
- Vitamin K deficiency prognosis
Vitamin K
Vitamin K is a fat-soluble vitamin that is naturally present in some foods and is available as a dietary supplement that is important for blood clotting and healthy bones and other diverse physiological functions 1. Vitamin K has thus been clinically applied for the treatment and prevention of bleeding and osteoporosis 2. Vitamin K is present in the diet in the forms of phylloquinone (vitamin K1) and menaquinones (vitamin K2) 3. Vitamin K1 (phylloquinone), which is the major dietary source, is concentrated in leafy vegetables (e.g., green vegetables) because it is directly involved in photosynthesis and is the vitamin K form best characterized in terms of food composition and dietary intakes. Vitamin K1 (phylloquinone) is active in animals and is responsible for the production of coagulation factors. Vitamin K1 (phylloquinone) is also can be converted into vitamin K2 (menaquinones) in animals 3. Vitamin K2 or menaquinones are the product of bacterial production or intestinal bacteria conversion from dietary vitamin K1 (phylloquinone) and are also found in fermented foods (e.g., cheeses and the Japanese soybean product natto [fermented soybeans]) (Figure 1) 4, 5. Vitamin K2 (menaquinones) have unsaturated isoprenyl side chains and are designated as MK-4 through MK-13, based on the length of their side chain 3, 6. MK-4, MK-7, and MK-9 are the most well-studied menaquinones. Food composition databases are limited for vitamin K2 (menaquinones) and their presence in foods varies by region. Dietary intakes of all forms of vitamin K vary widely among age groups and population subgroups. Similarly, the utilization of vitamin K from different forms and food sources appear to vary, although our understanding of vitamin K is still rudimentary in light of new developments regarding the vitamin K2 (menaquinones) 7.
Phylloquinone, also referred to as vitamin K1, is a compound present in all photosynthetic plants (e.g., green vegetables) and is the major dietary form of vitamin K in most diets 8. Vitamin K1 (phylloquinone) is the primary dietary source of vitamin K. Green leafy vegetables and some plant oils (soybean, canola, olive, and cottonseed) are major contributors of dietary vitamin K 9. Mixed dishes have also been found to significantly contribute to vitamin K intake in the US 10. However, vitamin K1 (phylloquinone) bioavailability from green vegetables is lower than from oil or supplements 9. Also, the phylloquinone content of green vegetables depends on their content in chlorophyll (green pigment), so that outer leaves have more phylloquinone than inner leaves 9. The efficiency of phylloquinone intestinal absorption varies among plant sources and is increased with the addition of a fat source to a meal. Finally, the hydrogenation of vegetable oils may decrease the absorption and biological effect of dietary phylloquinone 11, 12. A number of phylloquinone-rich foods are listed in Table 5, with their content in phylloquinone expressed in micrograms (μg). In general, green, leafy vegetables contain the highest known phylloquinone concentrations and contribute approximately 60% of total phylloquinone intake 5, 13. As indicated, spinach and collards, which have concomitant high concentrations of chlorophyll associated with the photosynthetic process, hence, dark leaf color have substantially higher concentrations of phylloquinone compared to the more commonly consumed iceberg lettuce, which is substantially paler, hence, lower chlorophyll concentrations. The other plant sources of phylloquinone are certain plant oils including soybean, canola (also known as rapeseed), cottonseed, and olive. Margarine, spreads, and salad dressings derived from these plant oils are important dietary sources of phylloquinone 14, 15. Plant oils are used for preparation of multiple mixed dishes, hence many commercially prepared foods including baked goods also contain small amounts of phylloquinone.
Menaquinones also known as vitamin K2, are the other category of vitamin K present in the food supply are primarily of bacterial origin and they are present in modest amounts in various animal-based and fermented foods (e.g., cheeses, curds, and the Japanese soybean product natto [fermented soybeans]) 11, 16. Almost all vitamin K2 (menaquinones), in particular the long-chain menaquinones (MK-n), are also produced by bacteria in the human gut 1, 17. Gut flora converts vitamin K1 (phylloquinone) into vitamin K2 (menaquinone). A range of vitamin K2 forms can be created. This transformation takes place via the gut bacteria lengthening the isoprenoid side chain. Bacteria are the primary producers of vitamin K2 (menaquinones), which they use during anaerobic respiration. They differ in structure from vitamin K1 (phylloquinone) due to the 3-substituted lipophilic side chain. The most important forms of vitamin K2 (menaquinones) contain 4 to 10 repeating isoprenoid units. These are indicated by MK-4 to MK-10. The most notable forms include MK-7 to MK-11. The MK-7 and other bacterially derived forms of vitamin K2 exhibit vitamin K activity in animals. Pharmacological doses of menaquinone-4 (MK-4; brand name, menatetrenone) are currently used in Japan in the treatment of osteoporosis 18. Accordingly, most intervention trials investigating the effect of high-dose MK-4 on bone loss have been conducted in Japanese postmenopausal women. In a three-year placebo-controlled trial among postmenopausal women with osteopenia, adding a MK-7 supplement (375 mcg/day) to combined calcium-vitamin D supplementation did not affect bone mineral density (BMD) or other bone health parameters despite reductions in serum undercarboxylated osteocalcin (ucOC) 19. At present, the potential role for supplemental menaquinones on bone health still needs to be established in large, randomized, and well-controlled trials.
The dominant dietary form of vitamin K in the United States and Europe is vitamin K1 (phylloquinone) (90% of daily intake), whereas the major form in Japan is vitamin K2 (menaquinones) (10%), especially menaquinone-7 (MK-7) 20. Vitamin K1 (phylloquinone) has a phythyl side chain, whereas vitamin K2 (menaquinones) comprises homologs that vary in the number (n) of isoprenyl groups of the side chain (from n = 1 to n = 14) 21. Among the vitamin K homologs, menaquinone-4 (MK-4) (n = 4) shows the greatest variety of physiological activities 22. Although vitamin K1 (phylloquinone) and menaquinone-4 (MK-4) have the same number of carbon atoms in the side chain, they differ in the degree of unsaturation. In Japan, vitamin K1 (phylloquinone) is applied as an antihemorrhagic agent and menaquinone-4 (MK-4) as a therapeutic agent for osteoporosis 2.
The review of five randomized clinical trials that assessed the effect of phylloquinone (vitamin K1) supplementation on hip bone mineral density (BMD) using doses ranging from 200 mcg/day to 5,000 mcg/day for durations of 12 to 36 months found little promising benefit for bone health 23. Although supplementation with phylloquinone decreased uncarboxylated osteocalcin (ucOC) levels in all five studies, only one study reported an effect of supplemental phylloquinone on bone mineral density (BMD) 18. In this study, 150 postmenopausal women were randomized to receive a placebo, minerals (500 mg/day of calcium, 150 mg/day of magnesium, and 10 mg/day of zinc) plus vitamin D (320 IU/day), or minerals, vitamin D, and phylloquinone (1,000 mcg/day) 18. The rate of BMD loss at the femoral neck, but not at the lumbar spine, was significantly lower in subjects with supplemental phylloquinone compared to the other two groups 18. Therefore, evidence of a putative benefit of phylloquinone on bone health in older adults is considered weak. None of the studies were designed to assess the effect of phylloquinone on osteoporotic-related fractures. Further investigation may seek to evaluate whether phylloquinone supplementation could improve skeletal health in subjects at high-risk for vitamin K inadequacy (e.g., individuals with malabsorption syndromes or cystic fibrosis).
Menadione, which is sometimes called “vitamin K3,” is another synthetic form of vitamin K. The synthetic vitamin K3 or menadione is very toxic, and as a result, has been banned from over-the-counter sales in the United States because ingestion could result in allergic reactions, hemolytic anemia, and cytotoxicity in liver cells 11. Vitamin K3 or menadione was shown to damage liver cells in laboratory studies conducted during the 1980s and 1990s, so it is no longer used in dietary supplements or fortified foods 24, 25. Vitamin K3 (menadione) can interfere with the function of glutathione, one of the body’s natural antioxidants, resulting in oxidative damage to cell membranes. Menadione (vitamin K3) given by injection has induced liver toxicity, jaundice, hyperbilirubinemia, and hemolytic anemia (due to the rupture of red blood cells) in infants and kernicterus in infants; therefore, menadione (vitamin K3) is no longer used for treatment of vitamin K deficiency 11, 26, 27, 16.
In the United States, vitamin K3 (menadione) is used in poultry feed and some swine feeds as a source of vitamin K 3. As such, menaquinone-4 (MK-4) formed from vitamin K3 (menadione) is present in poultry and pork products in the U.S. food supply and is the primary dietary source of MK-4 16. Menaquinone-4 (MK-4) is present at high concentrations in human, poultry and pork tissues 28. Although humans generally obtain vitamin K1 (phylloquinone) and menaquinone-7 (MK-7) from the diet, intake of menaquinone-4 (MK-4) in animal foods is extremely low. Vitamin K3 (menadione), a synthetic vitamin K analog (Figure 1), is the primary source of vitamin K in poultry feed and some swine feeds, along with small amounts of phylloquinone (vitamin K1) 3. As such, MK-4 formed from vitamin K3 (menadione) is present in poultry and pork products in the U.S. food supply and is the primary dietary source of MK-4 16. Although menaquinone-4 (MK-4) is also formed from tissue-specific conversion of phylloquinone (vitamin K1) 29, the impact on dietary intake from this conversion is likely negligible as animal organs containing high MK-4 concentrations including kidney, brain, and pancreas, are not commonly consumed in most regions of the world. Menaquinone-4 (MK-4) is also found in modest amounts in milk, butter, and cheeses, which may make a small contribution to total vitamin K intake. The high consumption of poultry, pork, and dairy products in the United States 30, however, suggests that MK-4 may make a relevant contribution to total vitamin K intake. In regions where food systems do not use vitamin K3 (menadione) in animal feed or consumption of dairy products is low, MK-4 is most likely not an important dietary source of vitamin K. For example, MK-4 has been estimated to account for ∼3% of total vitamin K intake in the Netherlands 31 and is found in animal products in relatively lower amounts compared with the United States and Japan 28.
Menaquinone-4 (MK-4) is unique among the vitamin K2 (menaquinones) in that it is produced by the body from vitamin K1 (phylloquinone) via a conversion process that does not involve bacterial action. Instead, menaquinone-4 (MK-4) is formed by a realkylation step from vitamin K3 (menadione) present in animal feeds or is the product of tissue-specific conversion directly from dietary vitamin K1 (phylloquinone) 32, 33, 34. In the United States, vitamin K3 (menadione) is the synthetic form of vitamin K used in poultry feed. As such, MK-4 formed from vitamin K3 (menadione) is present in poultry products in the US food supply 35. However, MK-4 formed from vitamin K1 (phylloquinone) is limited to organs not commonly consumed in the diet including kidney. The exceptions are dairy products with menaquinone-4 (MK-4) found in milk, butter, and cheese, albeit in modest amounts. Therefore it is unlikely that menaquinone-4 (MK-4) is an important dietary source of vitamin K in food supplies that do not use vitamin K3 (menadione) for poultry feed nor are rich in dairy products.
There is growing interest in the health benefits of longer-chain menaquinones (vitamin K2), which are limited to certain foods in the food supply. Menaquinone-7 (MK-7) is primarily the product of fermentation using bacillus subtilis natto and is present in a traditional Japanese soybean-based product called natto 11. Natto contains approximately 2.5 times more MK-7 compared to the vitamin K1 (phylloquinone) content of spinach. Natto also contains MK-8 and phylloquinone (84 and 35 µg/100g, respectively), although both are modest in concentration compared to MK-7 36. Some cheeses also contain MK-8 and MK-9 36, but these are dependent on cheese production practices, hence the food composition databases are limited in their ability to characterize vitamin K2 (menaquinone) intake across different food supplies.
Data on the bioavailability of different forms of vitamin K from food are very limited 3. The absorption rate of vitamin K1 (phylloquinone) in its free form is approximately 80%, but its absorption rate from foods is significantly lower 6. Vitamin K1 (phylloquinone) in plant foods is tightly bound to chloroplasts, so it is less bioavailable than that from oils or dietary supplements 3. For example, the body absorbs only 4% to 17% as much phylloquinone from spinach as from a tablet 6. Consuming vegetables at the same time as some fat improves vitamin K1 (phylloquinone) absorption from the vegetables, but the amount absorbed is still lower than that from oils. Limited research suggests that long-chain menaquinones (vtamin K2) may have higher absorption rates than phylloquinone from green vegetables 32.
Like dietary fats and other fat-soluble vitamins, ingested vitamin K is incorporated into mixed micelles via the action of bile and pancreatic enzymes, and it is absorbed by enterocytes of the small intestine 37. From there, vitamin K is incorporated into chylomicrons, secreted into the lymphatic capillaries, transported to the liver, and repackaged into very low-density lipoproteins 6. Vitamin K is present in the liver and other body tissues, including the brain, heart, pancreas, and bone 37.
In the circulation, vitamin K is carried mainly in lipoproteins 6. Compared to the other fat-soluble vitamins, very small amounts of vitamin K circulate in the blood. Vitamin K is rapidly metabolized and excreted. Based on phylloquinone measurements, the body retains only about 30% to 40% of an oral physiological dose, while about 20% is excreted in the urine and 40% to 50% in the feces via bile 38. This rapid metabolism accounts for vitamin K’s relatively low blood levels and tissue stores compared to those of the other fat-soluble vitamins 38.
Little is known about the absorption and transport of vitamin K produced by gut bacteria, but research indicates that substantial quantities of long-chain menaquinones (vitamin K2) are present in the large bowel 32. Although the amount of vitamin K that the body obtains in this manner is unclear, experts believe that these vitamin K2 (menaquinones) satisfy at least some of the body’s requirement for vitamin K 32.
Currently, there is not a consensus on a plasma vitamin K level indicating deficiency or insufficiency. Similarly, it is not clear which vitamer should be considered as reference for determining the vitamin K status 20. Data obtained in healthy subjects and osteoporotic patients supplemented with menaquinone-4 (MK-4) showed a large variability of vitamin K levels. In healthy subjects, levels of MK-4, vitamin K1 (phylloquinone) and MK-7 (reported as ng/mL and mean ± SD) were 0.15 ± 0.17, 1.81 ± 1.10 and 16.27 ± 20.58, respectively, while in osteoporotic patients receiving MK-4, these levels were 46.83 ± 46.41, 0.62 ± 0.25 and 4.18 ± 6.28, respectively 39. The influence of supplementation on menaquinone-4 (MK-4) levels was also observed in another study involving postmenopausal Japanese women 40, in contrast with the low MK-4 bioavailability reported in humans by Sato 41. Other authors measured vitamin K1 (phylloquinone) levels, demonstrating that vitamin K deficiency affects 24% of the general population and 29% of hemodialysis patients 42, 43. Vitamin K deficiency impairs blood coagulation process leading to issues with bleeding. Recent research has linked vitamin K deficiency to issues with osteoporosis and cystic fibrosis 44, 45, 11.
Total circulating levels of the bone protein, osteocalcin (OC), have been shown to be sensitive markers of bone formation 9. Several hormones and growth factors, including vitamin D but not vitamin K, regulate osteocalcin synthesis by osteoblasts 9. However, vitamin K is an essential cofactor for the gamma-carboxylation of three glutamic acid residues in osteocalcin 9. Undercarboxylation of osteocalcin (uncarboxylated osteocalcin [ucOC]) in human bone and serum has been linked to poor vitamin K status 9. The degree of osteocalcin gamma-carboxylation is responsive to vitamin K nutritional interventions, and thus is used as a relative indicator of vitamin K status 46.
Circulating levels of uncarboxylated osteocalcin (ucOC) were found to be higher in postmenopausal women than premenopausal women and markedly higher in women over the age of 70. Also, high ratios of uncarboxylated osteocalcin (ucOC) to total osteocalcin (OC) (ucOC/OC) appear to be predictive of hip fracture risk in elderly women 47, 48. Although vitamin K deficiency would seem the most likely cause of elevated blood uncarboxylated osteocalcin (ucOC) to total osteocalcin (OC) (ucOC/OC) ratio, some investigators have documented an inverse relationship between biochemical measures of vitamin D nutritional status and uncarboxylated osteocalcin (ucOC) levels, as well as a significant lowering of ucOC/OC ratio by vitamin D supplementation 49. It has been suggested that increased circulating ucOC/OC ratio could reflect a poor overall nutritional status that would include vitamin D deficiency, which would explain the above-mentioned observations 50. However, in several randomized, placebo-controlled intervention studies conducted in young girls 50, 51 or menaquinone-7 (vitamin K (2)): the Postmenopausal Health Study II. Calcif Tissue Int. 2012 Apr;90(4):251-62. doi: 10.1007/s00223-012-9571-z)) and postmenopausal women 52, vitamin D supplementation failed to decrease ucOC/OC ratios or show any additive effect on ucOC/OC lowering by supplemental vitamin K.
The monitoring of vitamin K administration or levels is usually through prothrombin time (PT) and INR (international normalized ratio). These values measure the presence of vitamin K-dependent factors, which is especially important to utilize in patients who have warfarin toxicity or vitamin K-related bleeding disorders (coagulopathies).
Figure 1. Vitamin K chemical structure
Footnote: Forms of vitamin K. (A) Menadione (vitamin K3) represents the basic structure common to vitamin K1 and vitamin K2; (B) phylloquinone (vitamin K1), which is the primary dietary source; (C) menaquinone-4 (MK-4), which is a conversion product from menadione (vitamin K3) or phylloquinone (vitamin K1); and (D) menaquinones (vitamin K2), which can vary in length from MK-4 to MK-13.
[Source 3 ]Figure 2. Vitamin K1 chemical structure
Figure 3. Vitamin K2 chemical structure
Are all forms of vitamin K the same?
There are surprisingly little data on the relative biological availability of different forms of vitamin K among different food sources. Furthermore, there is a growing body of literature to suggest that our understanding of vitamin K is still rudimentary in light of new developments regarding different forms 3.
As previously stated, vitamin K1 (phylloquinone) is of plant origin, with absolute intakes being predominantly from green leafy vegetables. Vitamin K1 (phylloquinone) is tightly bound to the membranes of plant chloroplasts, and is less bioavailable compared to phylloquinone obtained from plant oils and/or dietary supplements 53. Some estimates place the absorption of vitamin K1 (phylloquinone) to be 10% from plants compared to supplements 54. However there appear to be differences in absorption compared to the plant species, with vitamin K1 (phylloquinone) obtained from broccoli and collards having greater absorption compared to spinach 55. Similarly, and not unexpected because vitamin K is a fat-soluble vitamin, addition of a fat source to the meal results in higher absorption 38.
The commercial hydrogenation of phylloquinone-rich oils results in a transformation of phylloquinone into a hydrogenated form, 2′,3′-dihydrophylloquinone 56. Vitamin K1 (phylloquinone) differs from 2′,3′-dihydrophylloquinone by a saturation of a single bond at the 2′,3′ position of the side chain 56. Surprisingly this single substitution results in a lower absorption of dihydophylloquinone compared to an equimolar amount of phylloquinone 57. There is also indirect evidence of lower activity of dihydrophylloquinone as an enzyme cofactor, which currently is the only known function of vitamin K. The implications of this poor bioavailability and activity are currently unknown, although at least one study suggests a detrimental effect on bone mineral density among older adults in a community-based cohort 58. The potential impact of poor utilization of dihydrophylloquinone on bone health will be of little importance in the future should hydrogenated oils be removed from the food supply. It is anticipated that the decrease in hydrogenation of plant oils will also reduce the presence of dihydrophylloquinone in the US food supply 3.
Vitamin K2 (menaquinones) are poorly understood in terms of vitamin K absorption and utilization. MK-7, when administered in the form of natto in equimolar amounts to vitamin K1 (phylloquinone) administered in the form of spinach, has a peak height difference of more than 10-fold compared to phylloquinone, with a half-life of 56 hours, compared to 7.5 hours for vitamin K1 (phylloquinone) 59. Whereas all forms of vitamin K appear to be initially associated with triglyceride-rich lipoproteins, the longer chain menaquinones including MK-7 and MK-9 are also associated with low-density lipoprotein (LDL). MK-4 has been reported in triglyceride-rich lipoprotein, low-density lipoprotein (LDL) and high density lipoproteins (HDL). These preliminary data suggest that vitamin K2 (menaquinones) have different transport pathways and distribution, which has implications for transport to extra-hepatic tissue such as bone 38.
Emerging studies on MK-4 challenge our current understanding of vitamin K. As demonstrated using stable isotopes, MK-4 is a conversion product of vitamin K1 (phylloquinone) via the intermediate, vitamin K3 (menadione) 29. There appears to be both local and systemic conversion to MK-4, with the local conversion being the predominant pathway. The implications of this conversion are still the topic of speculation. To add complexity to the interpretation is the observation that this conversion does not occur in all tissues. Whereas the liver contains primarily vitamin K1 (phylloquinone) and very long-chain menaquinones, MK-4 is the predominant form in the brain, pancreas, and glands 60. In terms of dietary intakes, MK-4 intakes are low compared to other forms of vitamin K. However, dietary vitamin K1 (phylloquinone) converts to MK-4 in those tissues where MK-4 appears to be required so it is likely that low MK-4 intakes are of little consequence to health when there is adequate dietary vitamin K1 (phylloquinone) available for conversion to MK-4.
In conclusion, much of our understanding of vitamin K nutrition has focused on the primary dietary source, vitamin K1 (phylloquinone). There are comprehensive databases available that contain vitamin K1 (phylloquinone) contents of a variety of foods. Dietary assessment of phylloquinone reveals variation in intakes by age and population subgroups. In contrast, vitamin K2 (menaquinones) are present in the food supply, but there are limited food composition data available. As a corollary, estimates of intakes of vitamin K2 (menaquinones) are very limited and our understanding of their role in vitamin K nutrition is not well understood.
Vitamin K2
Vitamin K2 also known as menaquinones designated as MK-4 through MK-13 are the product of bacterial production or conversion from dietary vitamin K1 (phylloquinone) and are also found in fermented foods (e.g., cheeses and the Japanese soybean product natto) (Figures 1 and 3) 4, 5. Vitamin K2 (menaquinones) exist in multiple forms; however, the tendency in the literature to group all menaquinones under the term vitamin K2 has erroneously led many to assume that all vitamin K2 (menaquinones) are similar in origin and function. Menaquinones are primarily of bacterial origin, and differ in structure from vitamin K1 (phylloquinone) in their 3-substituted lipophilic side chain. The major vitamin K2 (menaquinones) contain 4–10 repeating isoprenyl side chains and are designated as MK-4 through MK-13, based on the length of their side chain 3, 6. MK-4, MK-7, and MK-9 are the most well-studied menaquinones. Food composition databases are limited for vitamin K2 (menaquinones) and their presence in foods varies by region. Dietary intakes of all forms of vitamin K vary widely among age groups and population subgroups. Similarly, the utilization of vitamin K from different forms and food sources appear to vary, although our understanding of vitamin K is still rudimentary in light of new developments regarding the vitamin K2 (menaquinones) 7.
Almost all vitamin K2 (Menaquinones) in particular the long-chain menaquinones are created in the gut by bacteria 1, 17. Gut flora converts vitamin K1 (phylloquinone) into vitamin K2 (menaquinone). A range of vitamin K2 forms can be created. This transformation takes place via the gut bacteria lengthening the isoprenoid side chain during anaerobic respiration. Vitamin K2 (menaquinones) differ in structure from vitamin K1 (phylloquinone) due to the 3-substituted lipophilic side chain. The most important forms of vitamin K2 (menaquinones) contain 4 to 10 repeating isoprenoid units. These are indicated by MK-4 to MK-10. In the human large intestine, the major forms of vitamin K2 (menaquinones) found to be present, including MK-6, MK7, MK-8, MK-10, and MK11, are produced by several types of enterobacteria such as Bacteroides, Enterobacteria, Eubacterium lentum, and Veillonella 61. Although intestinal bacteria synthesis is described to contribute to vitamin K requirements 62, it is not yet clear its true contribution to human vitamin K2 nutrition, and there is a need for further progress in this area 63. The MK-7 and other bacterially derived forms of vitamin K2 exhibit vitamin K activity in animals. The synthetic type of vitamin K, vitamin K3 (menadione), interferes with glutathione, which causes toxicity to animals. For this reason, vitamin K3 is no longer a viable treatment for vitamin K deficiency 3.
Menaquinone-4 (MK-4) is unique among the vitamin K2 (menaquinones) in that it is produced by the body from vitamin K1 (phylloquinone) via a conversion process that does not involve bacterial action. Instead, menaquinone-4 (MK-4) is formed by a realkylation step from vitamin K3 (menadione) present in animal feeds or is the product of tissue-specific conversion directly from dietary vitamin K1 (phylloquinone) 32, 33, 34. In the United States, vitamin K3 (menadione) is the synthetic form of vitamin K used in poultry feed. As such, MK-4 formed from vitamin K3 (menadione) is present in poultry products in the US food supply 35. However, MK-4 formed from vitamin K1 (phylloquinone) is limited to organs not commonly consumed in the diet including kidney. The exceptions are dairy products with menaquinone-4 (MK-4) found in milk, butter, and cheese, albeit in modest amounts. Therefore it is unlikely that menaquinone-4 (MK-4) is an important dietary source of vitamin K in food supplies that do not use vitamin K3 (menadione) for poultry feed nor are rich in dairy products.
There is growing interest in the health benefits of longer-chain menaquinones (vitamin K2), which are limited to certain foods such as dairy products, meats, and fermented foods 3. Menaquinone-7 (MK-7) is primarily the product of fermentation using bacillus subtilis natto and is present in a traditional Japanese soybean-based product called natto 11. Natto contains approximately 2.5 times more MK-7 compared to the vitamin K1 (phylloquinone) content of spinach. Natto also contains MK-8 and phylloquinone (84 and 35 µg/100g, respectively), although both are modest in concentration compared to MK-7 36. Some cheeses also contain MK-8 and MK-9 36, but these are dependent on cheese production practices, hence the food composition databases are limited in their ability to characterize vitamin K2 (menaquinone) intake across different food supplies.
What does Vitamin K2 do?
The primary function of vitamin K2 (menaquinone) is adding carboxylic acid groups (carboxylation) to glutamate (Glu) residues to form gamma-carboxyglutamate (Gla) residues during the creation of clotting factors (clotting factor II, VII, IX, X) (Figure 4 Vitamin K Cycle) 64. The presence of two carboxylic acid groups on a single carbon that resides in the gamma-carboxyglutamate (Gla) residue allows for the chelation of calcium ions. The binding of calcium ions in this fashion is of crucial importance for vitamin K-dependent clotting factors, permitting the perpetuation of the clotting cascades. Vitamin K is also responsible as a factor in synthesizing prothrombin, factor VII, factor IX, and factor X 65.
Carboxylation is catalyzed by the enzyme gamma-glutamyl carboxylase (GGCX), which utilizes a reduced form of vitamin K hydroquinone, carbon dioxide and oxygen as cofactors (Figure 4). Concomitant with each glutamate modification, vitamin K hydroquinone is oxidized to vitamin K 2,3-epoxide (vitamin K epoxide) 66. Vitamin K epoxide is converted back to vitamin K hydroquinone through a two-step reduction (first to vitamin K, then to vitamin K hydroquinone) using the enzymes vitamin K epoxide reductase (VKOR) and vitamin K reductase (VKR) in a pathway known as the vitamin K cycle (Figure 3). Importantly, warfarin interferes with regeneration of vitamin K 2,3-epoxide (vitamin K epoxide) to vitamin K hydroquinone, thus impairing gamma-carboxylation and the activity of vitamin K dependent proteins, including the extra-hepatic proteins Osteocalcin (bone Gla-protein) and matrix Gla-protein (MGP), respectively involved in bone mineralization and inhibition of vascular calcifications. If vitamin K is deficient, vitamin K dependent proteins cannot increase their carboxylation status (and they become significantly undercarboxylated), losing their capacity to bind calcium, so that bone metabolism may be impaired and the process of vascular calcification enhanced 67.
Vitamin K-dependent carboxylation was originally observed in clotting factors 68. In all clotting factors, 10 to 12 gamma-carboxyglutamate (Gla) residues are located in a homologous amino-terminal region referred to as the Gla domain 69. The multiple gamma-carboxyglutamate (Gla) residues in this domain adopt a calcium-dependent conformation that promotes clotting factors binding to a membrane surface 70, such as damaged vascular endothelial cells or activated platelets, which allows the localization of clotting factors near the site of vascular injury to either promote or regulate clotting.
With the discovery of new gamma-carboxyglutamate (Gla) proteins, vitamin K-dependent carboxylation has been implicated in a number of biological functions beyond coagulation. Osteocalcin is a Gla protein produced by osteoblasts and is important for bone formation 71. Recent studies suggest that osteocalcin also functions as a hormone affecting glucose metabolism in mice 72; whether this is the case in humans still needs to be clarified 73. The second Gla protein found in bone is matrix Gla protein (MGP), which functions as a strong inhibitor of vascular calcification and connective tissue mineralization 74. Defects of matrix Gla protein (MGP) carboxylation have been associated with cardiovascular diseases and pseudoxanthoma elasticum syndrome 75. In addition, MGP has been described as a critical regulator of endothelial cell function, regulating both physiological and tumor-related angiogenesis 76. Other vitamin K-dependent proteins that are not involved in coagulation include Gas6 (growth arrest-specific protein 6), PRGPs (proline-rich Gla proteins) and TMGs (transmembrane Gla proteins). In the nervous system, vitamin K2 (menaquinones) plays a crucial role in regulating many functions, such as sphingolipid synthesis 77, Growth arrest-specific protein 6 (Gas6)—associated-signaling 78 and cognition 79. These functions are associated with gamma-glutamyl carboxylase (GGCX). In the human brain, menaquinone-4 (MK-4) is the most abundant form of vitamin K 80. A better understanding of the structure-function relationship of the enzymes in the vitamin K cycle will help you to better understand and control a variety of biological processes (see Figure 3 below).
Lately, researchers have demonstrated that vitamin K is also involved in building bone. Low levels of circulating vitamin K have been linked with low bone density, and supplementation with vitamin K shows improvements in biochemical measures of bone health 81. There is a consistent line of evidence in human epidemiologic and intervention studies that clearly demonstrates that vitamin K can improve bone health. The human intervention studies have demonstrated that vitamin K can not only increase bone mineral density in osteoporotic people but also actually reduce fracture rates. Further, there is evidence in human intervention studies that vitamins K and D, a classic in bone metabolism, works synergistically on bone density 81. Several mechanisms are suggested by which vitamin K can modulate bone metabolism. Besides the gamma-carboxylation of osteocalcin, a protein believed to be involved in bone mineralization, there is increasing evidence that vitamin K also positively affects calcium balance, a key mineral in bone metabolism. The Institute of Medicine recently has increased the dietary reference intakes of vitamin K to 90 microg/d for females and 120 microg/d for males, which is an increase of approximately 50% from previous recommendations 81. A report from the Nurses’ Health Study suggests that women who get at least 110 micrograms of vitamin K a day are 30 percent less likely to break a hip than women who get less than that 6. Among the nurses, eating a serving of lettuce or other green, leafy vegetable a day cut the risk of hip fracture in half when compared with eating one serving a week. Data from the Framingham Heart Study also shows an association between high vitamin K intake and reduced risk of hip fracture in men and women and increased bone mineral density in women 82, 83.
Pharmacological doses of menaquinone-4 (MK-4; brand name, menatetrenone) are currently used in Japan in the treatment of osteoporosis 18. Accordingly, most intervention trials investigating the effect of high-dose MK-4 on bone loss have been conducted in Japanese postmenopausal women. In a three-year placebo-controlled trial among postmenopausal women with osteopenia, adding a MK-7 supplement (375 mcg/day) to combined calcium-vitamin D supplementation did not affect bone mineral density (BMD) or other bone health parameters despite reductions in serum undercarboxylated osteocalcin (ucOC) 19. At present, the potential role for supplemental menaquinones on bone health still needs to be established in large, randomized, and well-controlled trials.
A 2019 systematic review and meta-analysis of clinical trials in postmenopausal women or women with osteoporosis found vitamin K supplementation — of any form — lowered risk of clinical fracture compared to controls (9 trials varying from 6 months to 2 years: 3 using phylloquinone, 5 using MK-4, and 1 using MK-7) 84. However, no benefit of vitamin K supplementation was seen for vertebral fractures (7 trials) or bone mineral density (BMD) (22 trials) 84. The authors of this meta-analysis noted that the high heterogeneity of the included trials (i.e., form and dose of vitamin K, use of other supplements and drugs by participants, and treatment length) makes it difficult to inform clinical recommendations 84.
Vitamin K2 health benefits
Main vitamin K actions in humans include 20:
- Regulation of blood coagulation activity
- Bone protection; prevention of osteoporosis and bone fracture
- Prevention of vascular calcifications
- Prevention of cancer
- Prevention of inflammation
The primary function of vitamin K2 is adding carboxylic acid groups to glutamate residues (Glu) to form gamma-carboxyglutamate residues (Gla) during the creation of clotting factors 25. The presence of two carboxylic acid groups on a single carbon that resides in the gamma-carboxyglutamate residue (Gla) allows for the chelation of calcium ions. The binding of calcium ions in this fashion is of crucial importance for vitamin K-dependent clotting factors, permitting the perpetuation of the clotting cascades. Vitamin K is also responsible as a factor in synthesizing prothrombin, factor VII, factor IX, and factor X 44, 85.
Several studies suggest that low vitamin K levels are related to osteoporosis, pathological fractures and vascular calcifications 20. Supplementing menaquinone-7 (MK-7) at the dose of at least 200 mcg per day might help protecting from vascular calcification, osteoporosis and cancer 86. Moreover, supplementation of 5 mg daily phylloquinone (vitamin K1) in 440 postmenopausal women with osteopenia for 2 years in a randomized, placebo-controlled, double-blind trial caused a > 50% reduction in clinical fractures vs. placebo, although no protection against the age-related decline in bone mineral density was observed 87.
A meta-analysis has shown that in seven Japanese trials reporting fractures, vitamin K2 (menaquinones) administration significantly reduced the risk of hip (77% reduction), vertebral (60% reduction) and all non-vertebral fractures (81% reduction) 88. Vitamin K administration also significantly delayed the progression of coronary artery calcifications and the deterioration of arterial elasticity 89. A lower risk of coronary heart disease and severe aortic calcifications was observed with higher menaquinones intake, but not with phylloquinone intake. This finding suggests that the dietary phylloquinone intake, without menaquinones, may not be sufficient to suppress arterial calcifications 90.
Vitamin K2 (menaquinones) have also been shown to play an important role in cancer. In a small (40 patients) randomized study the administration of vitamin K2 (menaquinones) 45 mg/day reduced the development of hepatocellular carcinoma in patients with liver cirrhosis: the risk ratio for the development of hepatocellular carcinoma in patients given menaquinones was 0.13 91.
Vitamin K2 (menaquinones) have additional properties in certain cell and tissue types, particularly in bone tissue and in the immune system. Much of the available evidence relates specifically to menaquinone-4 (MK-4), which was found to have a role in bone health since the 1990s. Low circulating levels of vitamin K2 (menaquinones) are associated with osteoporotic fractures in the elderly 92 and vitamin K2 (menaquinones) improved bone mineral density in Japanese women 93. In an experimental setting, MK-4 reduced bone losses caused by either estrogen withdrawal or corticosteroid treatment in experimental model on rats 94, 95. Moreover, other in vitro studies showed that menaquinone-4 (MK-4) inhibits the synthesis of prostaglandin E2 (PGE2), a bone reabsorption-inducing agent, in cultured osteoblasts 96 and inhibits the formation of osteoclast-like cells in bone marrow-derived cultures 97. Finally, experimental data suggests a possible role of MK-4 on pancreatic exocrine cells metabolism. Stimulation of pancreatic acinar cells with secretagogues cholecystokinin-8 and secretin induces secretion of MK-4, along with phospholipase and the membrane trafficking protein caveolin-1 98, although a well-defined function of MK-4 in this setting remains unclear.
In the Vitamin K Italian (VIKI) study 99, a comprehensive assessment of vitamin K status was carried out in a cohort of hemodialysis patients and in healthy controls, including most vitamin K subtypes (in particular phylloquinone [vitamin K1], MK-4, MK-5, MK-6, and MK-7), adjusted for triglycerides levels. Vitamin K deficiency was found in 35.4% of hemodialysis patients for MK-7, 23.5% for PK and 14.5% for MK-4. With the limitations of its observational nature, this is the first study to relate vitamin K1 (phylloquinone) and vitamin K2 (menaquinones) deficiency directly both to vertebral fractures and vascular calcification in the dialysis population 99. In particular, vitamin K1 (phylloquinone) deficiency was the strongest predictor of vertebral fractures, while lower MK-4 and MK-7 levels were associated with vascular calcification. The results in hemodialysis patients may point out a possible role of vitamin K deficiency as a cause of bone and vascular disease also in the general population. It can be hypothesized that a diet rich in vitamin K and/or vitamin K supplements might be of help in preventing bone disease and avoiding vascular calcifications, opening interesting perspectives for research in human health.
The frequent use of warfarin enhances the problem of vitamin K deficiency and its role on bone and vascular disease 100. Warfarin may predispose to bone fractures and vascular calcification by different mechanisms: directly, by inhibition of gamma-carboxylation of osteocalcin and other bone matrix proteins; indirectly, because patients treated with warfarin may limit their dietary intake of foods rich in vitamin K. New oral anticoagulant seems to have less influence on bone metabolism, but their long-term effects need more studies 101, 102.
Finally, vitamin K status was found to be inversely and significantly related to individual inflammatory markers and to the inflammatory process in a human population study based on the Framingham Offspring Study cohort 103. This finding is supported by studies on rats demonstrating that animals with vitamin K-deficient diets had an enhanced expression of genes involved in acute inflammatory response compared to those with normal or phylloquinone-supplemented diets and that a supplemented diet suppressed the inflammatory response 104.
Bone health
Considerable attention has been given to the role of vitamin K in bone health, with an emphasis on vitamin K2 (menaquinones) 105. The biological basis for this has been the presence of vitamin K in bone and the dependence on vitamin K for carboxylation of multiple Gla-containing proteins synthesized in bone. The most notable of these proteins is osteocalcin, a vitamin K–dependent protein synthesized by osteoblasts during bone formation and the predominant noncollagenous protein in bone 106. Chronically low vitamin K intake results in suboptimal carboxylation of osteocalcin, which is thought to lead to decreased bone mineralization and increased risk of fracture and osteoporosis 107. However, the relationship of osteocalcin carboxylation status to bone health is unclear 105. More recently, some have proposed mechanisms that are independent of vitamin K’s role as an enzyme cofactor and that may be exclusive to MKs. Numerous in vitro and in vivo studies have been conducted, but overall, there is a lack of consensus regarding differential effects of vitamin K1 (phylloquinone) and vitamin K2 (menaquinones) on bone health 105.
In 2009, the European Food Safety Authority issued a scientific opinion concluding that “a cause and effect relationship has been established between the dietary intake of vitamin K and the maintenance of normal bone” 108. However, although both menaquinone-4 (MK-4) and menaquinone-7 (MK-7) supplementation consistently reduce the proportion of osteocalcin that is undercarboxylated 109, whether this effect favorably influences bone health is less clear 105. The first meta-analysis of MK-4 supplementation and bone health outcomes reported an overall benefit of MK-4 supplementation on reducing fracture risk 110. However, the authors articulated many caveats regarding their analysis, including geographic homogeneity, varied supplementation with other nutrients and medications, and heterogeneous study designs. In addition, the majority of these studies focused on MK-4 given therapeutically in daily doses of 45 mg, an amount unattainable through diet alone. A more recent meta-analysis combined findings of studies that used either vitamin K1 (phylloquinone) or vitamin K2 (menaquinones) supplementation and examined bone mineral density 111. The authors concluded that there were modest improvements in bone mineral density with vitamin K treatment, but that the results should be interpreted with caution, especially as vitamin K1 (phylloquinone), MK-4, and MK-7 were combined in the analysis, which assumes equivalent bioavailability. Findings from the 2 included studies in which MK-7 supplementation alone was examined were inconsistent with bone health benefits documented at MK-7 doses of 180 mcg/day over 1 year in 1 study 112, but not at 360 mcg/day over 1 year in another 113.
Several relevant studies have been published since these meta-analyses. In a large clinical trial of >4000 postmenopausal women, supplementation with MK-4 and calcium compared with calcium supplementation alone provided no additional protection against bone fracture over 3 years 114. Kanellakis et al. 115: the Postmenopausal Health Study II. Calcif Tissue Int. 2012 Apr;90(4):251-62. doi: 10.1007/s00223-012-9571-z)) examined bone markers during a 1-year regimen in which postmenopausal women were randomized to daily consumption of dairy products fortified with calcium, vitamin D3, and either no vitamin K, 100 mcg vitamin K1 (phylloquinone), or 100 μg MK-7. After 1 year, all 3 interventions improved total bone mineral density relative to a group receiving no intervention. Although no additional benefit attributable to vitamin K1 (phylloquinone) or MK-7 consumption was observed on total bone mineral density, both vitamin K1 (phylloquinone) and MK-7 were shown to have favorable effects on lumbar spine bone mineral density relative to the control and calcium + vitamin D groups. In the longest clinical trial of MK-7 supplementation conducted to date, Knapen et al. 116 assessed bone health and strength in >200 postpmenopausal women randomized to receive a supplement containing 180 mcg/day MK-7 or a placebo over 3 years. Site-specific effects of MK-7 were reported, with marginal attenuation of reductions in bone mineral content and bone mineral density observed at the lumbar spine and femoral neck. No effects of MK-7 supplementation were documented for the total hip, and the effects of MK-7 on bone strength indices were inconsistent. Limited evidence of favorable bone health effects when lower amounts of MK-7 are consumed from dietary sources also containing calcium and/or vitamin D suggests that the combination of nutrients rather than MK-7 alone may have greater efficacy in improving bone health 51 or menaquinone-7 (vitamin K (2)): the Postmenopausal Health Study II. Calcif Tissue Int. 2012 Apr;90(4):251-62. doi: 10.1007/s00223-012-9571-z)). In this regard, it is also important to note that, to date, there is no indication of greater efficacy of dietary vitamin K2 (menaquinones) relative to vitamin K1 (phylloquinone) 117. Furthermore, supplemental MK-7 has adverse effects on coagulation parameters in individuals receiving oral anticoagulant therapy 118. Therefore, any presumed benefit of MK-7 supplementation on bone health will need to be balanced with potential safety concerns regarding stability of oral anticoagulant therapy among patients on vitamin K–antagonists.
Anticancer activity
Although the mechanisms are still being sort out, in vitro (test tube) studies suggest that vitamin K2 (menaquinones) may arrest cell growth and induce apoptosis 119, 38, 120, 121, 122. A small number of in vivo (human) studies have investigated the efficacy of MK-4 supplementation in doses far in excess of what could be achieved in the diet for preventing hepatocellular carcinoma in high-risk patients. In a secondary analysis of a trial investigating MK-4 supplementation and bone loss, 45 mg/day of MK-4 was shown to reduce hepatocellular carcinoma risk in women with viral cirrhosis 123. However, results from subsequent studies investigating the efficacy of MK-4 supplementation for preventing hepatocellular carcinoma recurrence have been inconclusive 124. Vitamin K2 (menaquinones) have not been tested as an add-on cancer treatment. The most likely explanation for this is that vitamin K supplementation is contraindicated in cancer patients who are at increased risk of venous thromboembolism and are often prescribed vitamin K antagonists 125. Limited evidence is available to suggest that vitamin K intake reduces cancer risk in healthy adults. In 1 large prospective cohort, inverse relationships between dietary vitamin K2 (menaquinones) intake with incidence of prostate cancer, overall cancer incidence, and cancer mortality were reported 126. However, replication of these findings is needed.
Cardiovascular health
Calcification of vessel walls reduces their elasticity, increasing cardiovascular disease risk 127. There is growing recognition that oral anticoagulants such as warfarin may be associated with increased arterial calcification 128. Anticoagulants inhibit the vitamin K–dependent carboxylation of matrix-Gla protein (MGP) in vascular smooth-muscle cells 129. In its carboxylated form, MGP functions as a calcification inhibitor. As such, anticoagulants are thought to impair the calcium-regulating activity of MGP, thereby leading to increased calcium deposition in the vessel walls. The functional dependence of MGP on vitamin K and associations between anticoagulant use and calcification underpin current speculation that vitamin K may be involved in the progression of cardiovascular disease.
Only 1 randomized clinical trial examining vitamin K supplementation and coronary artery calcification has been published to date. In this trial 130, vitamin K1 (phylloquinone) supplementation reduced progression of existing coronary artery calcification, although there was no effect on incidence of coronary artery calcification. These findings are consistent with the proposed biological mechanism of matrix-Gla protein (MGP), which inhibits further calcification 131. In another study 132, postmenopausal women receiving a supplement containing vitamin K1 (phylloquinone) demonstrated better carotid artery compliance and elasticity after 3 years than those receiving a similar supplement with no vitamin K1 (phylloquinone). However, although suggestive, no direct measures of calcification were reported.
One would predict that the impact of vitamin K1 (phylloquinone) and vitamin K2 (menaquinones) supplementation on reducing progression of vascular calcification should be similar given that both forms support the gamma-carboxylation of matrix-Gla protein (MGP). However, a series of observational studies suggest possible differential effects of vitamin K2 (menaquinones) and vitamin K1 (phylloquinone) on arterial calcification and coronary heart disease risk. For example, inverse associations between vitamin K2 (menaquinones) intake and severe aortic calcification 133, 134, the risk of coronary heart disease 31 and the risk of coronary heart disease mortality and all-cause mortality 133 have been reported. In these studies, estimated vitamin K2 (menaquinones) intakes were up to 10 times lower than vitamin K1 (phylloquinone) intakes, and vitamin K1 (phylloquinone) intakes were not associated with disease risk. In the Dutch PROSPECT study cohort 28, the reduction in coronary heart disease risk was mainly driven by MK-7, MK-8, and MK-9 consumption.
Although these findings are intriguing and have stimulated considerable interest in a potential therapeutic role for vitamin K2 (menaquinones) in the progression of coronary artery calcification and coronary artery disease, these observational data should be interpreted with caution. Unlike vitamin K1 (phylloquinone), long-chain menaquinones are not detectable in circulation unless provided in high doses, either in the form of a supplement 135 or a concentrated food source, such as natto 136. Interestingly, MK-4 is often not detectable in the circulation, even after administration with doses as high as 420 mcg 135. This is problematic for validation of self-reported intakes of vitamin K2 (menaquinones) as there is no comparable biomarker. Use of vitamin K biomarkers that measure the proportion of carboxylation of vitamin K–dependent proteins reflect the dietary contribution of all forms of vitamin K and cannot be used to validate intakes of a single vitamin K2 (menaquinones). Because food composition databases for long-chain menaquinones are not available, it is unlikely that the epidemiological data can be replicated in other countries in the near future. Alternatively, the findings may reflect the use of vitamin K2 (menaquinones) as a marker of another nutrient or food constituent that has heart-healthy properties 137. For example, dairy products, which were the primary dietary source of vitamin K2 (menaquinones) in the Dutch PROSPECT study cohort, contain specific fatty acids that may confer protective effects on coronary artery disease risk 138. Notably an inverse association between coronary artery disease risk and the consumption of meat, milk, and cheese, among the richest sources for vitamin K2 (menaquinones) in the Dutch food supply, was also observed 31, 136. Given the challenges in interpreting the results of these studies, there is clearly a need for randomized clinical trials that isolate any putative effect of individual vitamin K2 (menaquinones) on coronary artery calcification. There have been recent media reports that nutritional doses of MK-7 (180 mcg/day) taken over a 3-years period prevented age-related arterial stiffening in postmenopausal women 139. However, the results of the clinical trial were not available in the peer-reviewed literature and the unique protective role of vitamin K2 (menaquinones) in heart health remains speculative 28.
How much vitamin K2 do I need?
The amount of vitamin K you need depends on your age and sex. Average daily recommended amounts are listed below in micrograms (mcg) (Table 1). The current US dietary guidelines for intakes of vitamin K are 90 and 120 mcg/day for women and men, respectively 140. These guidelines are termed adequate intakes (AI) because the Institute of Medicine concluded in 2001 that there were insufficient data available to generate a precise recommendation for vitamin K 82. The adequate intake (AI) values for vitamin K were generated from the Third National Health and Nutrition Examination Survey (NHANES III, 1988–1994) and based on the median vitamin K1 (phylloquinone) intake in the United States for each age and gender category 140. In the absence of abnormal bleeding associated with low vitamin K intakes among adults, it was assumed that the current intakes are adequate. However, the adequacy of intake defined by an absence of bleeding is controversial. Furthermore, the elderly report median intakes below the current adequate intake (AI) for adults. There is controversy regarding biochemical measures of subclinical vitamin K deficiency and as a consequence, the true dietary requirement of vitamin K is unknown 23. By comparison, the guidelines in the United Kingdom are 1 mcg/kg body weight per day 141 and are set at 75 mcg/day for adult men, 60 mcg/day for women, aged 18–29 year, and 65 mcg/day for women 30 years and over in Japan 142.
Very little is known about the contribution of dietary vitamin K2 (menaquinones) to overall vitamin K nutrition and although it has been stated that approximately 50% of the daily requirement for vitamin K is supplied by the gut flora through the production of vitamin K2 (menaquinones), there is little evidence to support this estimate 63. In one study among adults with acute bacterial overgrowth as induced by omeprazole, vitamin K2 (menaquinones) produced by these bacteria had some contribution to vitamin K status during dietary vitamin K1 (phylloquinone) restriction, but not enough to restore biochemical measures of vitamin K back to normal range 143.
Furthermore, there are regional differences in the forms and content of vitamin K2 (menaquinones) in the food supply. For example, natto is unique to a traditional Japanese diet whereas the cheeses that contain high concentrations of MK-8 and MK-9 appear to be most prevalent in European dairy producing food supplies 3. Although there are reported vitamin K2 (menaquinones) intakes, these are limited to studies from Japan 142 and the Netherlands 144 and are low compared to vitamin K1 (phylloquinone) intakes. In the United States, vitamin K2 (menaquinones) are limited in the food supply and have not been systematically assessed.
Table 1. Adequate Intakes for Vitamin K
Life Stage | Recommended Amount |
---|---|
Birth to 6 months | 2.0 mcg |
7–12 months | 2.5 mcg |
1–3 years | 30 mcg |
4–8 years | 55 mcg |
9–13 years | 60 mcg |
14–18 years | 75 mcg |
Adult men 19 years and older | 120 mcg |
Adult women 19 years and older | 90 mcg |
Pregnant or breastfeeding teens | 75 mcg |
Pregnant or breastfeeding women | 90 mcg |
Vitamin K2 food sources
Vitamin K2 (menaquinones) are primarily found in dairy products, meats, and fermented foods (Table 2) 3. In addition, egg yolks and high-fat dairy products, such as hard cheeses, provide appreciated amounts of vitamin K2 (menaquinones) 3. Of note, cheese was found to be the most important source of dietary long-chain menaquinones (MK-8 and MK-9) 146. In particular, propionibacteria-fermented cheese, such as Norwegian Jarlsberg cheese and Swiss Emmental cheese, were shown to have the highest concentration of vitamin K2 in the form of tetrahydromenaquinone-9 [MK-9(4H)] 147. Another important dietary source of vitamin K2 (menaquinones), with interest for the industry, are fermented plant foods, such as natto. Natto is a traditional Japanese soybean food produced by fermenting cooked soybean with Bacillus subtilis natto and considered one of the most relevant dietary sources of MK-7 (around 1000 mcg/100 g natto) 148. However, the vitamin K2 (menaquinones) and vitamin K1 (phylloquinone) contents of many food products are unknown 28.
Current knowledge indicates that dairy products are the primary contributors to dietary vitamin K2 (menaquinones) intake, and industrial application of vitamin K2 (menaquinones)-producing bacteria in fermented dairy products may provide an effective means of increasing vitamin K2 (menaquinones) in the food supply. Several lactic acid bacteria commonly used for making fermented food products, and generally recognized as safe (GRAS), have been used for the biosynthetic production of vitamin K2 (menaquinones) for the last few decades, with significant production amounts of menaquinones (MK-7 to MK-10) 149. Nevertheless, some genera of bacteria widely used in the food industry, including Lactobacillus and Streptococcus, have lost the functional ability to produce vitamin K2. Due to this, the vitamin K2 (menaquinones) content of food products using these bacteria is almost undetectable 150. A study examining the capacity of several bacterial strains to produce vitamin K compounds selected three strains of Lactococcus lactis ssp. cremoris, two strains of Lactococcus lactis ssp. lactis, and Leuconostoc lactis as high producers able to deliver more than 230 nmol/g dried cells of MK-7 to MK-10 149. In fact, several other bacterial species including Brevibacterium linens, Brochontrix thermosphacta, Hafnia alvei, Staphylococcus xylosus, Staphylococcus equorum, and Arthrobacter nicotinae, which are commonly used in industrial food fermentations, are well-known to produce several forms of vitamin K2, from MK-5 to MK-9, in different amounts 28.
The specific bacterial strains used and production conditions during fermentation (i.e., pH, temperature, duration) likely determine the concentrations and forms of vitamin K2 (menaquinones) found in fermented food products. For example, tetrahydromenaquinone [MK-9(4H)], produced by propionibacteria and formed from partial hydrogenation of the isoprenoid chain in MK-9 151, has been measured in different varieties of cheese in which propionibacteria are used in starter cultures 147. High concentrations of MK-8 and MK-9 measured in Edam type cheese 152 is consistent with the use of the MK-8– and MK-9–producing lactic acid bacteria strains Lactococcus lactis ssp. lactis and L. lactis ssp. cremoris as starter cultures for these cheeses. However, few studies have used validated HPLC methods to quantify vitamin K2 (menaquinones) in fermented foods 150. The available literature shows that MK-8 and MK-9 are the most common bacterially synthesized vitamin K2 (menaquinones) found in fermented dairy products, with the presence of other vitamin K2 (menaquinones) being more limited (Table 1). In a recent report, total long-chain menaquinone concentrations ranged from nondetectable to 118 mcg/100 g, with a median concentration of 15 mcg/100 g in 62 European fermented dairy products 150. Long-chain menaquinones have also been measured in fermented plant-based foods such as sauerkraut and natto, a fermented soybean product popular in certain regions of Japan but not widely consumed elsewhere 142. The long-chain menaquinone contents of meat and fish products are generally low 142 and likely have little public health importance. As such, the evidence available to date suggests that dairy products are likely the predominant dietary sources of long-chain menaquinones. In support of this claim, cheese and milk products were estimated to contribute to 54% and 22% of total vitamin K2 (menaquinones) intake, respectively, in a cohort of Dutch women in whom long-chain menaquinones were estimated to account for 9% of total vitamin K intake 134. However, the absence of comprehensive data on food vitamin K2 (menaquinones) contents and regional differences in dairy consumption patterns indicate that much more research is needed to accurately quantify vitamin K2 (menaquinones) intake at the individual and population levels 28.
Table 2. Vitamin K2 (menaquinones) concentration in dairy foods and fermented food products
Vitamin K2 (menaquinones) 2 | |||||||
---|---|---|---|---|---|---|---|
Food | MK-4 | MK-5 | MK-6 | MK-7 | MK-8 | MK-9 | MK-10 |
Milk (mcg/100 mL) | |||||||
Whole | 0.8 | 0.1 | ND | ND–2.04 | ND | ND | ND |
Buttermilk | 0.2 | 0.1 | 0.1 | 0.1 | 0.6 | 1.4 | ND |
Yogurt (mcg/100 g) | |||||||
Whole | 0.6–1.0 | 0.1–0.3 | ND–0.2 | ND–0.4 | 0.2–2.0 | ND–4.7 | ND |
Skimmed | ND | ND | ND | ND | ND–0.1 | ND | ND |
Cheese (mcg/100 g) | |||||||
Curd | 0.4 | 0.1 | 0.2 | 0.3 | 5.1 | 18.7 | ND |
Hard | 4.7–10.2 | 1.5 | ND–3.0 | ND–2.3 | ND–16.9 | ND–51.1 | ND–6.5 |
Semihard | NR | NR | 1.0–3.5 | ND–2.1 | 2.5–7.3 | 10.0–32.1 | ND–13.8 |
Soft | 3.7 | 0.3 | 0.4–2.6 | ND–1.7 | 2.1–14.0 | 6.6–94.0 | ND–5.7 |
Other (mcg/100 g) | |||||||
Salami | 9 | ND | ND | ND | ND | ND | ND |
Sauerkraut | 0.4 | 0.8 | 1.5 | 0.2 | 0.8 | 1.1 | ND |
Natto | ND–2.0 | 7.5 | 13.8 | 939–998 | 84.1 | ND | ND |
Footnotes:
2 Values represent the mean concentration (if 1 study), or lowest and highest mean concentrations (if ≥2 studies or foods within the same category) reported in representative studies from the United States, Europe and Japan. Studies reported values as a range or mean of multiple samples for each food type. MK 11–13 concentrations were not reported in any study.
3 In mcg/100 mL.
4 In mcg/100 g.
Abbreviations: MK = menaquinone; ND = not detectable; NR = not reported.
[Source 28 ]Vitamin K2 side effects
Currently, there is no known toxicity is associated with high doses of vitamin K1 (phylloquinone) or vitamin K2 (menaquinones) 64. Therefore, there is no designated Tolerable Upper Intake Level (maximum daily intake unlikely to cause adverse health effects) 24. Despite this, an allergic reaction is possible with either version of vitamin K. Vitamin K1 has had documented associations with bronchospasm and cardiac arrest with IV administration. The oral form of vitamin K does not seem to cause severe reactions 153.
Vitamin K2 also does not display any adverse effects when ingested orally. Studies have shown that coagulation studies in humans did not show an increased risk of blood clots when ingesting 45 mg per day of vitamin K2 (as MK-4). Researchers observed this in a patient who took upwards of 135 mg per day (45 mg three times per day) 154, 155.
What does Vitamin K do?
Main vitamin K actions in humans include 20:
- Regulation of blood coagulation activity
- Bone protection; prevention of osteoporosis and bone fracture
- Prevention of vascular calcifications
- Prevention of cancer
- Prevention of inflammation
Vitamin K functions as a coenzyme for vitamin K-dependent carboxylase, an enzyme required for the synthesis of proteins involved in hemostasis (blood clotting) and bone metabolism, and other diverse physiological functions 24, 1. Vitamin K helps make four of the 13 proteins needed for blood clotting. Vitamin K is required in the synthesis of 4 clotting factors in the liver: factors II,VII, IX, and X. Vitamin K is also essential in the production of protein C and S, which are anticoagulant proteins 156.
Prothrombin (clotting factor II) is a vitamin K-dependent protein in plasma that is directly involved in blood clotting. Its role in maintaining the clotting cascade is so important that people who take anticoagulants such as warfarin (Coumadin) must be careful to keep their vitamin K intake stable. Warfarin (Coumadin®) and some anticoagulants used primarily in Europe antagonize the activity of vitamin K and, in turn, prothrombin 157. For this reason, individuals who are taking these anticoagulants need to maintain consistent vitamin K intakes. Coumarin anticoagulants interfere with the synthesis of vitamin–K dependent coagulation proteins (factors II, VII, IX, and X) in the liver.
Carboxylation is catalyzed by the enzyme gamma-glutamyl carboxylase (GGCX), which utilizes a reduced form of vitamin K hydroquinone, carbon dioxide and oxygen as cofactors (Figure 2). Concomitant with each glutamate modification, vitamin K hydroquinone is oxidized to vitamin K 2,3-epoxide (vitamin K epoxide) 66. Vitamin K epoxide is converted back to vitamin K hydroquinone through a two-step reduction (first to vitamin K, then to vitamin K hydroquinone) using the enzymes vitamin K epoxide reductase (VKOR) and vitamin K reductase (VKR) in a pathway known as the vitamin K cycle (Figure 4). Importantly, warfarin interferes with regeneration of vitamin K 2,3-epoxide (vitamin K epoxide) to vitamin K hydroquinone, thus impairing gamma-carboxylation and the activity of vitamin K dependent proteins, including the extra-hepatic proteins Osteocalcin (bone Gla-protein) and matrix Gla-protein (MGP), respectively involved in bone mineralization and inhibition of vascular calcifications. Matrix Gla-protein (MGP), a vitamin K-dependent protein present in vascular smooth muscle, bone, and cartilage, is the focus of considerable scientific research because it might help reduce abnormal calcification 1, 133, 158. Osteocalcin (OC) is another vitamin K-dependent protein that is present in bone and may be involved in bone mineralization or turnover 1.
If vitamin K is deficient, vitamin K dependent proteins cannot increase their carboxylation status and they become significantly undercarboxylated, losing their capacity to bind calcium, so that bone metabolism may be impaired and the process of vascular calcification enhanced 159.
With the discovery of new gamma-carboxyglutamate (Gla) proteins, vitamin K-dependent carboxylation has been implicated in a number of biological functions beyond coagulation. Osteocalcin is a Gla protein produced by osteoblasts and is important for bone formation 71. Recent studies suggest that osteocalcin also functions as a hormone affecting glucose metabolism in mice 72; whether this is the case in humans still needs to be clarified 73. The second Gla protein found in bone is matrix Gla protein (MGP), which functions as a strong inhibitor of vascular calcification and connective tissue mineralization 74. Matrix Gla-protein (MGP), a vitamin K-dependent protein present in vascular smooth muscle, bone, and cartilage, is the focus of considerable scientific research because it might help reduce abnormal calcification 160. Defects of matrix Gla protein (MGP) carboxylation have been associated with cardiovascular diseases and pseudoxanthoma elasticum syndrome 75. In addition, MGP has been described as a critical regulator of endothelial cell function, regulating both physiological and tumor-related angiogenesis 76. Other vitamin K-dependent proteins that are not involved in coagulation include Gas6 (growth arrest-specific protein 6), PRGPs (proline-rich Gla proteins) and TMGs (transmembrane Gla proteins). The metabolic significance of most of these non-coagulation Gla proteins is still poorly understood 65. A better understanding of the structure-function relationship of the enzymes in the vitamin K cycle will help you to better understand and control a variety of biological processes.
Lately, researchers have demonstrated that vitamin K is also involved in building bone. Low levels of circulating vitamin K have been linked with low bone density, and supplementation with vitamin K shows improvements in biochemical measures of bone health 81. There is a consistent line of evidence in human epidemiologic and intervention studies that clearly demonstrates that vitamin K can improve bone health. The human intervention studies have demonstrated that vitamin K can not only increase bone mineral density in osteoporotic people but also actually reduce fracture rates. Further, there is evidence in human intervention studies that vitamins K and D, a classic in bone metabolism, works synergistically on bone density 81. Several mechanisms are suggested by which vitamin K can modulate bone metabolism. Besides the gamma-carboxylation of osteocalcin, a protein believed to be involved in bone mineralization, there is increasing evidence that vitamin K also positively affects calcium balance, a key mineral in bone metabolism. The Institute of Medicine recently has increased the dietary reference intakes of vitamin K to 90 microg/d for females and 120 microg/d for males, which is an increase of approximately 50% from previous recommendations 81. A report from the Nurses’ Health Study suggests that women who get at least 110 micrograms of vitamin K a day are 30 percent less likely to break a hip than women who get less than that 6. Among the nurses, eating a serving of lettuce or other green, leafy vegetable a day cut the risk of hip fracture in half when compared with eating one serving a week. Data from the Framingham Heart Study also shows an association between high vitamin K intake and reduced risk of hip fracture in men and women and increased bone mineral density in women 82, 83.
Table 3. Consequences of inhibition of vitamin K-dependent proteins
Coagulation | Prothrombin (factor II) VII, IX, X factors Protein C, S, Z | Bleeding |
Blood vessels | MGP Osteocalcin GAS-6 | Vascular calcifications |
Bone | Osteocalcin MGP Periostin | Inadequate bone mineralization Bone fractures |
Cellular proliferation | GAS-6 | Action on cellular proliferation, cellular adhesion, inhibition of apoptosis |
Inflammation | Not defined | Increase of inflammation |
Abbreviations: MGP = matrix Gla protein; BGP = bone Gla protein; GAS = growth arrest specific gene
[Source 20 ]Vitamin K cycle
Vitamin K obtained from the diet is considered to reach the target tissues via lipid absorption and the transport system 161. Once transferred to the cells of the target tissue, vitamin K is metabolized by redox (reduction–oxidation) cycling in the intracellular endoplasmic reticulum body, in a process known as the “vitamin K cycle”, “vitamin K oxidation-reduction cycle” or “vitamin K-epoxide cycle” (Figure 4) 162. This series of oxidation-reduction reactions begins with conversion of vitamin K from a stable oxidized form (quinone form [KO]) to a vitamin K hydroquinone (reduced form [KH2]) by vitamin K-epoxide reductase (VKOR) 163, 164. Gamma-glutamyl carboxylase (GGCX) carboxylates the glutamic acid residues of vitamin K-dependent proteins (VKDP) to gamma-carboxyglutamate (Gla) using reduced vitamin K (vitamin K hydroquinone), while simultaneously oxidizing the reduced form of vitamin K to a vitamin K epoxide (oxidized form). These reactions that gamma-glutamyl carboxylase (GGCX) catalyzes proceed on the GGCX protein molecule using CO2 and O2; however, the detailed molecular mechanisms are not clear. The epoxide form of vitamin K (oxidized form) is reduced by epoxide reductase (vitamin K epoxide reductase complex 1; VKORC1 or vitamin K epoxide reductase complex 1-like 1; VKORC1L1) to a reduced form and then to the reduced hydroquinone form 165. This reuse system allows for a very small amount of vitamin K in cells to act efficiently as a cofactor of gamma-glutamyl carboxylase (GGCX) in the post-translational carboxylation of vitamin K-dependent proteins (VKDPs). Warfarin, an oral anticoagulant drug, inhibits vitamin K epoxide reductase (VKOR), stops the vitamin K cycle, and prevents the gamma-glutamyl-carboxylated (Gla) conversion of the blood coagulation factors, thus inhibiting coagulation (Figure 4). Activity of both gamma-glutamyl carboxylase (GGCX) and vitamin K epoxide reductase (VKOR) are regulated by calumenin 166. Recent evidence has revealed that GGCX is the only enzyme involved in Gla formation, based on structure and function analyses of GGCX at the gene level and animal studies showing that GGCX gene deficiency causes embryonic lethality from systemic bleeding.
Some oral anticoagulants, such as warfarin (Jantoven, formerly known as Coumadin), inhibit coagulation by inhibiting the action of vitamin K. Warfarin prevents the recycling of vitamin K by blocking vitamin K-epoxide reductase (VKOR) activity, hence preventing vitamin K recycling and therefore creating a functional vitamin K deficiency (Figure 4) 9. Inadequate gamma-carboxylation of vitamin K-dependent coagulation proteins interferes with the coagulation cascade, which inhibits blood clot formation. Large quantities of dietary or supplemental vitamin K can overcome the anticoagulant effect of vitamin K antagonists; thus, patients taking oral anticoagulants are cautioned against consuming very large or highly variable quantities of vitamin K (see Drug interactions). Experts now advise a reasonably constant dietary intake of vitamin K that meets current dietary recommendations (90-120 mcμg/day) for patients taking vitamin K antagonists like warfarin 12, 167.
Vitamin K acts as a cofactor for gamma-glutamyl carboxylase (GGCX) via the vitamin K cycle and exerts physiological effects through its regulation of vitamin K-dependent proteins (VKDPs) 168. More than 20 VKDPs have been found. Osteocalcin promotes bone formation, and blood coagulation factors II, VII, IX, and X activate blood coagulation. Matrix Gla protein suppresses cardiovascular calcification, and brain-expressed Gas 6 (growth arrest-specific protein 6) promotes neural differentiation for signaling and cognitive functions in the brain 169, 168. Gamma-glutamyl carboxylase (GGCX) is an enzyme that converts glutamic acid (Glu) residues to gamma-carboxyglutamate (Gla) residues, so that the Gla-containing proteins can exert various physiological actions such as blood coagulation and bone formation.
Few studies, however, have addressed derivatives targeting GGCX. Vermeer et al. 170 found increased gamma-glutamyl carboxylase (GGCX) activity with modifying the side-chain structure of vitamin K to a saturated alkyl side chain with an amide bond. Further modification of the side-chain structure is anticipated from synthesis of vitamin K derivatives that yield stronger GGCX activity. Development of such new derivatives may lead to drug discovery that can enhance the physiological effects associated with GGCX and other factors 171.
Figure 4. Vitamin K oxidation-reduction cycle
Footnotes: During vitamin K-dependent carboxylation, glutamate (Glu) is converted to gamma-carboxyglutamte (Gla) by gamma-glutamyl carboxylase (GGCX) using a reduced form of vitamin K hydroquinone, carbon dioxide, and oxygen as cofactors. Vitamin K hydroquinone is oxidized to vitamin K epoxide. Vitamin K epoxide is reduced to vitamin K by vitamin K epoxide reductase (VKOR). The reduction of vitamin K to vitamin K hydroquinone is carried out by vitamin K epoxide reductase (VKOR) and an as-yet-unidentified vitamin K reductase (VKR).
[Source 2 ]Vitamin K derivatives targeting steroid and xenobiotic receptor (SXR)
Both vitamin K1 (phylloquinone) and vitamin K2 (menaquinones) may activate the steroid and xenobiotic receptor (SXR) 20. Steroid and xenobiotic receptor (SXR) is a nuclear receptor involved in the transcriptional regulation of enzymes such as cytochrome P450 (in particular the CYP3A4 isoform) 172.
Tabb et al. 173 revealed that menaquinone-4 (MK-4) regulates gene expression as a ligand of the nuclear receptor SXR. Steroid and xenobiotic receptor (SXR) is mainly expressed in the liver and intestine and regulates expression of genes encoding enzymes involved in steroid metabolism and detoxification of xenobiotics and of various drugs 173. When bound to a ligand, SXR forms a heterodimer with retinoid X receptor, and the resulting complex then binds to an SXR responsive element on the target gene promoter via the DNA-binding domain to exert transcription regulation 174. In addition to bile acids (e.g., lithocholic acid), drugs such as rifampicin, SR12813, and hyperforin are ligands involved in this process. Among the vitamin K homologs, menaquinone-4 (MK-4) can activate transcription of the SXR target gene CYP3A4, as a ligand of SXR 174. MK-4 plays an important role in osteoblast formation by inducing expression of genes such as matrilin-2 and tsukushi, which are involved in collagen accumulation via SXR 175. An in vitro study further showed that overexpression of SXR and its activation by MK-4 inhibits proliferation and migration of liver cancer cells 176. More recently, the data showed that vitamin K2 (menaquinone) has a differentiation-promoting effect on myeloid progenitors and an anti-apoptotic effect on erythroid progenitors 177.
Thus, menaquinone-4 (MK-4) works via SXR to regulate the expression of various genes at the transcriptional level, resulting in broad physiological effects such as bone formation and liver cancer suppression as well as drug metabolism 2. However, to date, MK-4 is the only vitamin K homolog known to exert its activities via SXR, and further research is needed to clarify whether other vitamin K homologs act as SXR ligands. X-ray crystal structure analysis of complexes of human pregnane X receptor (PXR) and ligands (such as rifampicin) have demonstrated that the ligand-binding region of SXR is large, with substantial flexibility 178, suggesting that other vitamin K congeners likely could act as SXR ligands.
Because menaquinone-4 (MK-4) is present in the brain at a relatively high concentration, it is thought to have important roles in the brain 179. Vitamin K protects neural cells from oxidative stress; however, a crucial role for vitamin K in the brain has not been elucidated 2. Neural stem cells engage in continuous self-replication while maintaining the ability to differentiate into neurons and glial cells in the early embryonic and late fetal stages. Neural stem cells can differentiate into neuronal precursor cells and glial precursor cells, and each progenitor cell differentiates into neurons, astrocytes, and oligodendrocytes. The neural stem cells that do not differentiate into neurons differentiate into glial cells before and after birth, at which point differentiation into neurons is complete 180.
Neuronal progenitor cells differentiate into neurons, while glial progenitor cells differentiate into astrocytes and oligodendrocytes 181. Scientists recently reported that vitamin K2 (menaquinone) show weak activity in driving the differentiation of progenitor cells to neuronal cells 182, which depended on the number of isoprene units of the side chain in the vitamin K homolog. If this differentiation activity can be increased by modification of the chemical structure, it would be possible to regulate it with a specific neuronal differentiation inducer based on a low-molecular-weight compound. Such a compound could provide an alternate strategy to conventional gene induction methods used in induced pluripotent stem cells and other types of stem and progenitor cells. Researchers are focusing on the role of vitamin K in the regeneration of neurons, and synthesized vitamin K analogs that could differentiate progenitor cells into neuronal cells.
Are you getting enough vitamin K?
In most cases, vitamin K status is not routinely assessed, except in individuals who take anticoagulants or have bleeding disorders. The only clinically significant indicator of vitamin K status is prothrombin time (the time it takes for blood to clot), and ordinary changes in vitamin K intakes have rarely been shown to alter prothrombin time 1. In healthy people, fasting concentrations of phylloquinone (vitamin K1) in plasma have been reported to range from 0.29 to 2.64 nmol/L 183. However, it is not clear whether this measure can be used to quantitatively assess vitamin K status. People with plasma phylloquinone concentrations slightly below the normal range have no clinical indications of vitamin K deficiency, possibly because plasma phylloquinone concentrations do not measure the contribution of menaquinones from the diet and the large bowel 183. No data on normal ranges of menaquinones (vitamin K2) are available 6.
Vitamin K deficiency is very rare. People who do not regularly eat a lettuce salad or green, leafy vegetables are likely to be deficient in their intake of vitamin K; national data suggests that only about one in four Americans meets the goal for vitamin K intake from food 184. The current US dietary guidelines for intakes of vitamin K are 90 and 120 µg/day for women and men, respectively 185.
Most people in the United States get enough vitamin K from the foods they eat. Also, bacteria in the colon make some vitamin K that the body can absorb. However, certain groups of people may have trouble getting enough vitamin K:
- Newborns who don’t receive an injection of vitamin K at birth
- People with conditions (such as cystic fibrosis, celiac disease, ulcerative colitis, and short bowel syndrome) that decrease the amount of vitamin K their body absorbs
- People who have had bariatric (weight loss) surgery
What happens if you don’t get enough vitamin K?
Severe vitamin K deficiency can cause bruising and bleeding problems because the blood will take longer to clot. Vitamin K deficiency might reduce bone strength and increase the risk of getting osteoporosis because the body needs vitamin K for healthy bones.
Several studies suggest that low vitamin K levels are related to osteoporosis, pathological fractures and vascular calcifications 20. Supplementing menaquinone-7 (MK-7) at the dose of at least 200 mcg per day might help protecting from vascular calcification, osteoporosis and cancer 86. Moreover, supplementation of 5 mg daily phylloquinone (vitamin K1) in 440 postmenopausal women with osteopenia for 2 years in a randomized, placebo-controlled, double-blind trial caused a > 50% reduction in clinical fractures vs. placebo, although no protection against the age-related decline in bone mineral density was observed 87.
A meta-analysis has shown that in seven Japanese trials reporting fractures, vitamin K2 (menaquinones) administration significantly reduced the risk of hip (77% reduction), vertebral (60% reduction) and all non-vertebral fractures (81% reduction) 88. Vitamin K administration also significantly delayed the progression of coronary artery calcifications and the deterioration of arterial elasticity 89. A lower risk of coronary heart disease and severe aortic calcifications was observed with higher menaquinones intake, but not with phylloquinone intake. This finding suggests that the dietary phylloquinone intake, without menaquinones, may not be sufficient to suppress arterial calcifications 90.
Vitamin K2 (menaquinones) have been shown to play an important role also in cancer. In a small (40 patients) randomized study the administration of menaquinones 45 mg/day reduced the development of hepatocellular carcinoma in patients with liver cirrhosis: the risk ratio for the development of hepatocellular carcinoma in patients given menaquinones was 0.13 91.
Vitamin K2 (menaquinones) have additional properties in certain cell and tissue types, particularly in bone tissue and in the immune system. Much of the available evidence relates specifically to menaquinone-4 (MK-4), which was found to have a role in bone health since the 1990s. Low circulating levels of vitamin K2 (menaquinones) are associated with osteoporotic fractures in the elderly 92 and vitamin K2 (menaquinones) improved bone mineral density in Japanese women 93. In an experimental setting, MK-4 reduced bone losses caused by either estrogen withdrawal or corticosteroid treatment in experimental model on rats 94, 95. Moreover, other in vitro studies showed that menaquinone-4 (MK-4) inhibits the synthesis of prostaglandin E2 (PGE2), a bone reabsorption-inducing agent, in cultured osteoblasts 96 and inhibits the formation of osteoclast-like cells in bone marrow-derived cultures 97. Finally, experimental data suggests a possible role of MK-4 on pancreatic exocrine cells metabolism. Stimulation of pancreatic acinar cells with secretagogues cholecystokinin-8 and secretin induces secretion of MK-4, along with phospholipase and the membrane trafficking protein caveolin-1 98, although a well-defined function of MK-4 in this setting remains unclear.
In the Vitamin K Italian (VIKI) study 99, a comprehensive assessment of vitamin K status was carried out in a cohort of hemodialysis patients and in healthy controls, including most vitamin K subtypes (in particular phylloquinone [vitamin K1], MK-4, MK-5, MK-6, and MK-7), adjusted for triglycerides levels. Vitamin K deficiency was found in 35.4% of hemodialysis patients for MK-7, 23.5% for PK and 14.5% for MK-4. With the limitations of its observational nature, this is the first study to relate vitamin K1 (phylloquinone) and vitamin K2 (menaquinones) deficiency directly both to vertebral fractures and vascular calcification in the dialysis population 99. In particular, vitamin K1 (phylloquinone) deficiency was the strongest predictor of vertebral fractures, while lower MK-4 and MK-7 levels were associated with vascular calcification. The results in hemodialysis patients may point out a possible role of vitamin K deficiency as a cause of bone and vascular disease also in the general population. It can be hypothesized that a diet rich in vitamin K and/or vitamin K supplements might be of help in preventing bone disease and avoiding vascular calcifications, opening interesting perspectives for research in human health.
The frequent use of warfarin enhances the problem of vitamin K deficiency and its role on bone and vascular disease 100. Warfarin may predispose to bone fractures and vascular calcification by different mechanisms: directly, by inhibition of gamma-carboxylation of osteocalcin and other bone matrix proteins; indirectly, because patients treated with warfarin may limit their dietary intake of foods rich in vitamin K. New oral anticoagulant seems to have less influence on bone metabolism, but their long-term effects need more studies 101, 102.
Finally, vitamin K status was found to be inversely and significantly related to individual inflammatory markers and to the inflammatory process in a human population study based on the Framingham Offspring Study cohort 103. This finding is supported by studies on rats demonstrating that animals with vitamin K-deficient diets had an enhanced expression of genes involved in acute inflammatory response compared to those with normal or phylloquinone-supplemented diets and that a supplemented diet suppressed the inflammatory response 104.
Vitamin K Health Benefits
Scientists are studying vitamin K to understand how it affects our health. Here are some examples of what this research has shown. However, the US Food and Drug Administration (FDA) has not authorized any health claims for any forms of vitamin K.
Osteoporosis
Osteoporosis, a disorder characterized by porous and fragile bones, is a serious public health problem that affects more than 10 million U.S. adults, 80% of whom are women. Consuming adequate amounts of calcium and vitamin D, especially throughout childhood, adolescence, and early adulthood, is important to maximize bone mass and reduce the risk of osteoporosis 186. The effect of vitamin K intakes and status on bone health and osteoporosis has been a focus of scientific research 187, 188, 189.
Vitamin K is a cofactor for the gamma-carboxylation of many proteins, including osteocalcin, one of the main proteins in bone 190. Some research indicates that high serum levels of undercarboxylated osteocalcin are associated with lower bone mineral density 1, 190. Some, but not all, studies also link higher vitamin K intakes with higher bone mineral density and/or lower hip fracture incidence 191, 192, 193, 194, 195, 196.
Although vitamin K is involved in the carboxylation of osteocalcin, it is unclear whether supplementation with any form of vitamin K reduces the risk of osteoporosis. In 2006, Cockayne and colleagues conducted a systematic review and meta-analysis of randomized controlled trials that examined the effects of vitamin K supplementation on bone mineral density and bone fracture 197. Most of the trials were conducted in Japan and involved postmenopausal women; trial duration ranged from 6 to 36 months. Thirteen trials were included in the systematic review, and 12 showed that supplementation with either phytonadione or MK-4 improved bone mineral density. Seven of the 13 trials also had fracture data that were combined in a meta-analysis. All of these trials used MK-4 at either 15 mg/day (1 trial) or 45 mg/day (6 trials). MK-4 supplementation significantly reduced rates of hip fractures, vertebral fractures, and all nonvertebral fractures.
A subsequent clinical trial found that MK-7 supplementation (180 mcg/day for 3 years) improved bone strength and decreased the loss in vertebral height in the lower thoracic region of the vertebrae in postmenopausal women 198. Other randomized clinical trials since the 2006 review by Cockayne et al. have found that vitamin K supplementation has no effect on bone mineral density in elderly men or women 199, 200. In one of these studies, 381 postmenopausal women received either 1 mg phylloquinone, 45 mg MK-4, or placebo daily for 12 months 200. All participants also received daily supplements containing 630 mg calcium and 400 IU vitamin D3. At the end of the study, participants receiving either phylloquinone or MK-4 had significantly lower levels of undercarboxylated osteocalcin compared to those receiving placebo. However, there were no significant differences in bone mineral density of the lumbar spine or proximal femur among any of the treatment groups. The authors noted the importance of considering the effect of vitamin D on bone health when comparing the results of vitamin K supplementation studies, especially if both vitamin K and vitamin D (and/or calcium) are administered to the treatment group but not the placebo group. The administration of vitamin D and/or calcium along with vitamin K could partly explain why some studies have found that vitamin K supplementation improves bone health while others have not.
In Japan and other parts of Asia, a pharmacological dose of MK-4 (45 mg) is used as a treatment for osteoporosis 1. The European Food Safety Authority has approved a health claim for vitamin K, noting that “a cause and effect relationship has been established between the dietary intake of vitamin K and the maintenance of normal bone” 201. The FDA has not authorized a health claim for vitamin K in the United States.
Vitamin K antagonists and bone health
Certain oral anticoagulants, such as warfarin, are known to be antagonists of vitamin K. Few studies have examined chronic use of warfarin and risk of fracture in older women. One study reported no association between long-term warfarin treatment and fracture risk 202, while another one found a significantly higher risk of rib and vertebral fractures in warfarin users compared to nonusers 203. Additionally, a study in elderly patients with atrial fibrillation reported that long-term warfarin treatment was associated with a significantly higher risk of osteoporotic fracture in men but not in women 204. A meta-analysis of the results of 11 published studies found that oral anticoagulation therapy was associated with a very modest reduction in bone mineral density (BMD) at the wrist and no change in bone mineral density (BMD) at the hip or spine 205. The development of new anticoagulants that do not block vitamin K recycling may offer a safer alternative to the use of vitamin K antagonists 206.
Osteoarthritis
Osteoarthritis, a degenerative joint condition that affects more than 32 million US adults 207, is characterized by the breakdown of articular cartilage (i.e., cartilage within the joint). Because several vitamin K-dependent proteins are present in cartilage and in bone 208, vitamin K deficiency may have a role in the development of osteoarthritis. A few observational studies have investigated a possible link between vitamin K intake or status and osteoarthritis. A cross-sectional study among 719 Japanese older adults found dietary intake of vitamin K to be inversely associated with knee osteoarthritis 209. In the Framingham Offspring Study (n=672; mean age, 66 years), higher plasma concentrations of phylloquinone were associated with a lower risk of hand, but not knee, osteoarthritis 210. A longitudinal study of 1,180 US adults (mean age, 62 years) found that low plasma concentrations of phylloquinone (≤0.5 nM) at baseline — indicative of a subclinical vitamin K deficiency — were associated with a 56% increase in risk of knee osteoarthritis after 30 months compared to those with higher plasma concentrations 211. In a more recent longitudinal study among 523 older US adults participating in the Health, Aging, and Body Composition Study, those with extremely low plasma concentrations of phylloquinone (<0.2 nM) at baseline had increased progression of knee osteoarthritis over three years, assessed by MRI of articular cartilage and the meniscus; those with higher plasma concentrations experienced no significant progression of knee osteoarthritis 212. Moreover, recent studies have associated use of the vitamin K antagonist drugs (e.g., warfarin and acenocoumarol) with higher risks of osteoarthritis and joint replacement of the knee and hip compared to nonusers 213.
While these observational data are interesting, randomized controlled trials are needed to determine whether vitamin K supplementation in those with low vitamin K status might help prevent or treat osteoarthritis. In an ancillary study of a double-blind, controlled trial examining the effects of vitamin K supplementation on bone loss and vascular calcification in older adults, no effects of phylloquinone supplementation (500 mcg/day) were found on incidence of hand osteoarthritis after three years 214. Study participants were not screened for vitamin K status, and in a subgroup analysis, those with serum phylloquinone ≤1 nM at baseline that reached >1 nM at year 3 had less joint deterioration. These data infer that only those individuals with low vitamin K status benefit from vitamin K supplementation. Unfortunately, no measures were available for knee osteoarthritis, and it is well established that hand and knee osteoarthritis represent different phenotypes. Additional clinical trials that specifically examine the effect of vitamin K supplementation on osteoarthritis development are needed, especially in those with inadequate vitamin K status.
Coronary heart disease
Scientists are studying whether low blood levels of vitamin K increase the risk of heart disease, perhaps by making blood vessels that feed the heart stiffer and narrower. More research is needed to understand whether vitamin K supplements might help prevent heart disease.
Vascular calcification is one of the risk factors for coronary heart disease because it reduces aortic and arterial elasticity 215. Matrix Gla-protein is a vitamin K-dependent protein that may play a role in the prevention of vascular calcification 1, 216. Although the full biological function of matrix Gla-protein is unclear, a hypothesis based on animal data suggests that inadequate vitamin K status leads to undercarboxylated matrix Gla-protein, which could increase vascular calcification and the risk of coronary heart disease. These findings might be particularly relevant for patients with chronic kidney disease because their rates of vascular calcification are much higher than those of the general population 160.
In an observational study conducted in the Netherlands in 564 postmenopausal women, dietary menaquinone (but not phylloquinone) intake was inversely associated with coronary calcification 217. Menaquinone intake was also inversely associated with severe aortic calcification in a prospective, population-based cohort study involving 4,807 men and women aged 55 years and older from the Netherlands 216. Participants in this study who had dietary menaquinone intakes in the mid tertile (21.6–32.7 mcg/day) and upper tertile (>32.7 mcg/day) also had a 27% and 57% lower risk of coronary heart disease mortality, respectively, than those in the lower tertile of intake (<21.6 mcg/day). Phylloquinone intake had no effect on any outcome.
Despite these data, few trials have investigated the effects of vitamin K supplementation on arterial calcification or coronary heart disease risk. One randomized, double-blind clinical trial examined the effect of phylloquinone supplementation in 388 healthy men and postmenopausal women aged 60–80 years 218. Participants received either a multivitamin (containing B-vitamins, vitamin C, and vitamin E) plus 500 IU vitamin D3, 600 mg calcium, and 500 mcg phylloquinone daily (treatment) or a multivitamin plus calcium and vitamin D3 only (control) for 3 years. There was no significant difference in coronary artery calcification between the treatment and control groups. However, among the 295 participants who adhered to the supplementation protocol, those in the treatment group had significantly less coronary artery calcification progression than those in the control group. Furthermore, among those with coronary artery calcification at baseline, phylloquinone treatment reduced calcification progression by 6% compared to the control group. Based on these findings, the authors did not make any clinical recommendations, and they called for larger studies in other populations 218.
At this time, the role of the different forms of vitamin K on arterial calcification and the risk of coronary heart disease is unclear, but it continues to be an active area of research in the general population and in patients with chronic kidney disease 1, 160, 219.
How much vitamin K do you need?
The amount of vitamin K you need depends on your age and sex. Average daily recommended amounts are listed below in micrograms (mcg).
Table 4. Adequate Intakes for Vitamin K
Life Stage | Recommended Amount |
---|---|
Birth to 6 months | 2.0 mcg |
7–12 months | 2.5 mcg |
1–3 years | 30 mcg |
4–8 years | 55 mcg |
9–13 years | 60 mcg |
14–18 years | 75 mcg |
Adult men 19 years and older | 120 mcg |
Adult women 19 years and older | 90 mcg |
Pregnant or breastfeeding teens | 75 mcg |
Pregnant or breastfeeding women | 90 mcg |
What foods provide vitamin K?
Food sources of vitamin K1 (phylloquinone) include vegetables, especially green leafy vegetables, vegetable oils, and some fruits. Meat, dairy foods, and eggs contain low levels of vitamin K1 (phylloquinone) but modest amounts of vitamin K2 (menaquinones) 16. Natto (a traditional Japanese food made from fermented soybeans) has high amounts of vitamin K2 (menaquinones) 3, 136. Other fermented foods, such as cheese, also contain vitamin K2 (menaquinones). However, the forms and amounts of vitamin K in these foods likely vary depending on the bacterial strains used to make the foods and their fermentation conditions 28. Animals synthesize MK-4 from menadione (vitamin K3) (a synthetic form of vitamin K that can be used in poultry and swine feed) 220. Thus, poultry and pork products contain MK-4 if menadione (vitamin K3) is added to the animal feed 28.
The most common sources of vitamin K in the U.S. diet are spinach; broccoli; iceberg lettuce; and fats and oils, particularly soybean and canola oil 1, 32. Few foods are fortified with vitamin K 1; breakfast cereals are not typically fortified with vitamin K, although some meal replacement shakes and bars are.
Vitamin K is found naturally in many foods. You can get recommended amounts of vitamin K by eating a variety of foods, including the following:
- Green leafy vegetables, such as spinach, kale, broccoli, and lettuce
- Vegetable oils
- Some fruits, such as blueberries and figs
- Meat, cheese, eggs, and soybeans
Although multiple databases now exist that contain some phylloquinone contents of foods 36, 221, the most extensive analysis of phylloquinone in common foods using established food sampling protocols 222 are found in the United States Department of Agriculture (USDA) Nutrient Database for Standard Reference 223.
The U.S. Department of Agriculture’s (USDA’s) FoodData Central website (https://fdc.nal.usda.gov) lists the nutrient content of many foods vitamin K (phylloquinone) arranged by nutrient content (https://ods.od.nih.gov/pubs/usdandb/VitK-Phylloquinone-Content.pdf) and by food name (https://ods.od.nih.gov/pubs/usdandb/VitK-Phylloquinone-Food.pdf), and of foods containing vitamin K (menaquinone) arranged by nutrient content (https://ods.od.nih.gov/pubs/usdandb/VitK-Menaquinone-Content.pdf) and food name (https://ods.od.nih.gov/pubs/usdandb/VitK-Menaquinone-Food.pdf).
Data on the bioavailability of different forms of vitamin K from food are very limited 224. The absorption rate of phylloquinone in its free form is approximately 80%, but its absorption rate from foods is significantly lower. Phylloquinone in plant foods is tightly bound to chloroplasts, so it is less bioavailable than that from oils or dietary supplements 224. For example, the body absorbs only 4% to 17% as much phylloquinone from spinach as from a tablet 6. Consuming vegetables at the same time as some fat improves phylloquinone absorption from the vegetables, but the amount absorbed is still lower than that from oils. Limited research suggests that long-chain MKs may have higher absorption rates than phylloquinone from green vegetables 32.
Several food sources of vitamin K are listed in Table 5. All values in this table are for phylloquinone content, except when otherwise indicated, because food composition data for menaquinones are limited 224.
Table 5. Selected Food Sources of Vitamin K (Phylloquinone)
Food | Micrograms (mcg) per serving | Percent DV* |
---|---|---|
Natto, 3 ounces (as MK-7) | 850 | 1062 |
Collards, frozen, boiled, ½ cup | 530 | 662 |
Turnip greens, frozen, boiled ½ cup | 426 | 532 |
Spinach, raw, 1 cup | 145 | 181 |
Kale, raw, 1 cup | 113 | 141 |
Broccoli, chopped, boiled, ½ cup | 110 | 138 |
Soybeans, roasted, ½ cup | 43 | 54 |
Carrot juice, ¾ cup | 28 | 34 |
Soybean oil, 1 tablespoon | 25 | 31 |
Edamame, frozen, prepared, ½ cup | 21 | 26 |
Pumpkin, canned, ½ cup | 20 | 25 |
Pomegranate juice, ¾ cup | 19 | 24 |
Okra, raw, ½ cup | 16 | 20 |
Salad dressing, Caesar, 1 tablespoon | 15 | 19 |
Pine nuts, dried, 1 ounce | 15 | 19 |
Blueberries, raw, ½ cup | 14 | 18 |
Iceberg lettuce, raw, 1 cup | 14 | 18 |
Chicken, breast, rotisserie, 3 ounces (as MK-4) | 13 | 17 |
Grapes, ½ cup | 11 | 14 |
Vegetable juice cocktail, ¾ cup | 10 | 13 |
Canola oil, 1 tablespoon | 10 | 13 |
Cashews, dry roasted, 1 ounce | 10 | 13 |
Carrots, raw, 1 medium | 8 | 10 |
Olive oil, 1 tablespoon | 8 | 10 |
Ground beef, broiled, 3 ounces (as MK-4) | 6 | 8 |
Figs, dried, ¼ cup | 6 | 8 |
Chicken liver, braised, 3 ounces (as MK-4) | 6 | 8 |
Ham, roasted or pan-broiled, 3 ounces (as MK-4) | 4 | 5 |
Cheddar cheese, 1½ ounces (as MK-4) | 4 | 5 |
Mixed nuts, dry roasted, 1 ounce | 4 | 5 |
Egg, hard boiled, 1 large (as MK-4) | 4 | 5 |
Mozzarella cheese, 1½ ounces (as MK-4) | 2 | 3 |
Milk, 2%, 1 cup (as MK-4) | 1 | 1 |
Salmon, sockeye, cooked, 3 ounces (as MK-4) | 0.3 | 0 |
Shrimp, cooked, 3 ounces (as MK-4) | 0.3 | 0 |
Footnote: *DV = Daily Value. The U.S. Food and Drug Administration (FDA) developed DVs to help consumers compare the nutrient contents of foods and dietary supplements within the context of a total diet. The DV for vitamin K is 120 mcg for adults and children age 4 years and older 225. FDA does not require food labels to list vitamin K content unless vitamin K has been added to the food. Foods providing 20% or more of the DV are considered to be high sources of a nutrient, but foods providing lower percentages of the DV also contribute to a healthful diet.
[Sources 16, 136, 226 ]Table 6. Vitamin K content of common foods
Food | Major form of vitamin K | Concentration (mcg/100g) |
---|---|---|
Vegetables | ||
Collards | Phylloquinone | 440 |
Spinach | Phylloquinone | 380 |
Broccoli | Phylloquinone | 180 |
Cabbage | Phylloquinone | 145 |
Iceberg lettuce | Phylloquinone | 35 |
Fats and oils | ||
Soybean oil | Phylloquinone | 193 |
Canola oil | Phylloquinone | 127 |
Cottonseed oil | Phylloquinone | 60 |
Olive oil | Phylloquinone | 55 |
Mixed dishes a | ||
Fast food french fries | Dihydrophylloquinone | 59 |
Fast food nachos | Dihydrophylloquinone | 60 |
Frozen, breaded fish sticks | Dihydrophylloquinone | 16 |
Margarine with hydrogenated oil | Dihydrophylloquinone | 102 |
Other foods | ||
Natto | Menaquinone-7 (MK-7) | 998 |
Hard cheeses | Menaquinone-9 (MK-9) | 51.1 |
Soft cheeses | Menaquinone-9 (MK-9) | 39.5 |
Footnote:
a These data reflect the content when hydrogenated phylloquinone-rich oils are used. When non-hydrogenated oils are used, the predominant form would be phylloquinone.
[Source 3 ]Vitamin K Supplements
Vitamin K is present in most multivitamin or multimineral supplements, typically at values less than 75% of the Daily Value (DV) 227. It is also available in dietary supplements containing only vitamin K or vitamin K combined with a few other nutrients, frequently calcium, magnesium, and/or vitamin D. These supplements tend to have a wider range of vitamin K doses than multivitamin/mineral supplements, with some providing 4,050 mcg (5,063% of the DV) or another very high amount.
Several forms of vitamin K are used in dietary supplements, including vitamin K1 as phylloquinone or phytonadione (a synthetic form of vitamin K1) and vitamin K2 as MK-4 or MK-7 227. Few data are available on the relative bioavailability of the various forms of vitamin K supplements. One study found that both phytonadione and MK-7 supplements are well absorbed, but MK-7 has a longer half-life 228.
Menadione, which is sometimes called “vitamin K3,” is another synthetic form of vitamin K. It was shown to damage liver cells in laboratory studies conducted during the 1980s and 1990s, so it is no longer used in dietary supplements or fortified foods 24, 25. Vitamin K3 (menadione) can interfere with the function of glutathione, one of the body’s natural antioxidants, resulting in oxidative damage to cell membranes. Menadione (vitamin K3) given by injection has induced liver toxicity, jaundice, hyperbilirubinemia, and hemolytic anemia (due to the rupture of red blood cells) in infants and kernicterus in infants; therefore, menadione (vitamin K3) is no longer used for treatment of vitamin K deficiency 11, 26, 27, 16.
Vitamin K Side Effects and Toxicity
Currently, there is no known toxicity is associated with high doses of vitamin K1 (phylloquinone) or vitamin K2 (menaquinones) 64. Therefore, the Food and Nutrition Board did not establish Tolerable Upper Intake Level (maximum daily intake unlikely to cause adverse health effects) for vitamin K because of its low potential for toxicity 24. In its report, the Food and Nutrition Board stated that “no adverse effects associated with vitamin K consumption from food or supplements have been reported in humans or animals” 24.
Despite this, an allergic reaction is possible with either version of vitamin K. Vitamin K1 has had documented associations with bronchospasm and cardiac arrest with IV administration. The oral form of vitamin K does not seem to cause severe reactions 153.
Vitamin K2 also does not display any adverse effects when ingested orally. Studies have shown that coagulation studies in humans did not show an increased risk of blood clots when ingesting 45 mg per day of vitamin K2 (as MK-4). Researchers observed this in a patient who took upwards of 135 mg per day (45 mg three times per day) 154, 155.
The only reported toxicity comes from vitamin K3 (menadione), which has no use in humans 25. Vitamin K3 (menadione) can interfere with the function of glutathione, one of the body’s natural antioxidants, resulting in oxidative damage to cell membranes. Menadione (vitamin K3) given by injection has induced liver toxicity, jaundice, hyperbilirubinemia, and hemolytic anemia (due to the rupture of red blood cells) in infants and kernicterus in infants; therefore, menadione (vitamin K3) is no longer used for treatment of vitamin K deficiency 11, 26, 27, 16.
Can vitamin K be harmful?
Vitamin K has not been shown to cause any harm. The Food and Nutrition Board at the Institute of Medicine of the National Academies did not establish Tolerable Upper Intake Level (maximum daily intake unlikely to cause adverse health effects) for vitamin K because of its low potential for toxicity 24. In its report, the Food and Nutrition Board stated that “no adverse effects associated with vitamin K consumption from food or supplements have been reported in humans or animals” 24. Although allergic reactions are possible, there is no known toxicity associated with high doses (dietary or supplemental) of the phylloquinone (vitamin K1) or menaquinone (vitamin K2) forms of vitamin K 24. The same is not true for synthetic menadione (vitamin K3) and its derivatives 24. Vitamin K3 (menadione) can interfere with the function of glutathione, one of the body’s natural antioxidants, resulting in oxidative damage to cell membranes. Menadione (vitamin K3) given by injection has induced liver toxicity, jaundice, hyperbilirubinemia, and hemolytic anemia (due to the rupture of red blood cells) in infants and kernicterus in infants; therefore, menadione (vitamin K3) is no longer used for treatment of vitamin K deficiency 11, 26, 27, 16.
Vitamin K and childhood leukemia
In the early 1990s, two retrospective studies were published suggesting a possible association between phylloquinone injections in newborns and the development of childhood leukemia and other forms of childhood cancer. However, two large retrospective studies in the US and Sweden, which reviewed the medical records of 54,000 and 1.3 million children, respectively, found no evidence of a relationship between childhood cancers and phylloquinone injections at birth 229, 230. Moreover, a pooled analysis of six case-control studies, including 2,431 children diagnosed with childhood cancer and 6,338 cancer-free children, found no evidence that phylloquinone injections for newborns increased the risk of childhood leukemia 231. In a policy statement, the American Academy of Pediatrics recommended that routine vitamin K prophylaxis for newborns be continued because vitamin K deficiency bleeding (VKDB) is life threatening and the risks of cancer are unproven and unlikely 232. In the last decade, physicians have reported a rise in late-onset cases of vitamin K deficiency bleeding (VKDB) due to an increasing trend of parents omission or refusal of newborn vitamin K prophylaxis 233, 234.
Lower doses of vitamin K1 for premature infants
The results of two studies of vitamin K levels in premature infants suggest that the standard initial dose of phylloquinone (vitamin K1) for full-term infants (1.0 mg) may be too high for premature infants 235, 236. These findings have led some experts to suggest the use of an initial phylloquinone dose of 0.3 mg/kg for infants with birth weights of less than 1,000 g (2 lbs, 3 oz), and an initial phylloquinone dose of 0.5 mg would probably prevent hemorrhagic disease in newborns 235. Yet, additional studies are needed to determine the best vitamin K prophylaxis in premature infants 237.
Vitamin K interactions with medications
Vitamin K interacts with a few medications. In addition, certain medications can have an adverse effect on vitamin K levels. Some examples are provided below. Individuals taking these and other medications on a regular basis should discuss their vitamin K status with their doctors. The use of cholesterol-lowering medications like cholestyramine (Questran®) and colestipol (Colestid®), as well as orlistat, mineral oil, and the fat substitute, olestra, may interfere with fat absorption and affect the absorption of fat-soluble vitamins, including vitamin K 238.
Warfarin (Coumadin®) and similar anticoagulants
Vitamin K can have a serious and potentially dangerous interaction with anticoagulants (vitamin K antagonists) such as warfarin (Coumadin®), as well as phenprocoumon, acenocoumarol, and tioclomarol, which are commonly used in some European countries 32. These drugs antagonize the activity of vitamin K, leading to the depletion of vitamin K-dependent clotting factors. People taking warfarin and similar anticoagulants need to maintain a consistent intake of vitamin K from food and supplements because sudden changes in vitamin K intakes can increase or decrease the anticoagulant effect 239. Moreover, daily phylloquinone supplements of up to 100 mcg are considered safe for patients taking warfarin, but therapeutic anticoagulant stability may be undermined by daily doses of MK-7 as low as 10 to 20 mcg 240. It is generally recommended that individuals using warfarin try to consume the adequate intake (90 mcg/day for women and 120 mcg/day for men) level for vitamin K (90-120 mcg/day) and avoid large fluctuations in vitamin K intake that might interfere with the adjustment of their anticoagulant dose 12, 167, 241. The prescription of anti-vitamin K anticoagulants, anticonvulsants (e.g., phenytoin), and anti-tuberculosis drugs (e.g., rifampin and isoniazid) to pregnant or breast-feeding women may place the newborn at increased risk of vitamin K deficiency 242.
Amiodarone
The drug amiodarone, used in the management of certain cardiac arrhythmias (irregular heartbeat), including atrial fibrillation, can enhance the anticoagulant effect of warfarin and thus increase the risk of hemorrhage 243, 244.
Antibiotics
Antibiotics can destroy vitamin K-producing bacteria in the gut, potentially decreasing vitamin K status. This effect might be more pronounced with cephalosporin antibiotics, such as cefoperazone (Cefobid®), because these antibiotics might also inhibit the action of vitamin K in the body 17. Vitamin K supplements are usually not needed unless antibiotic use is prolonged (beyond several weeks) and accompanied by poor vitamin K intake.
Bile acid sequestrants
Bile acid sequestrants, such as cholestyramine (Questran®) and colestipol (Colestid®), are used to reduce cholesterol levels by preventing reabsorption of bile acids. They can also reduce the absorption of vitamin K and other fat-soluble vitamins, although the clinical significance of this effect is not clear 245. Vitamin K status should be monitored in people taking these medications, especially when the drugs are used for many years 245.
Orlistat
Orlistat is a weight-loss drug that is available as both an over-the-counter (Alli®) and prescription (Xenical®) medication. It reduces the body’s absorption of dietary fat and in doing so, it can also reduce the absorption of fat-soluble vitamins, such as vitamin K. Combining orlistat with warfarin therapy might cause a significant increase in prothrombin time 246. Otherwise, orlistat does not usually have a clinically significant effect on vitamin K status, although clinicians usually recommend that patients taking orlistat take a multivitamin supplement containing vitamin K 247.
Vitamin A and vitamin E
Large doses of vitamin A and vitamin E have been found to antagonize vitamin K 248. Excess vitamin A appears to interfere with vitamin K absorption, whereas vitamin E may inhibit vitamin K-dependent carboxylase activity and interfere with the coagulation cascade 249. One study in adults with normal coagulation status found that supplementation with 1,000 IU/day of vitamin E for 12 weeks decreased gamma-carboxylation of prothrombin, a vitamin K-dependent protein 250. Individuals taking anticoagulatory drugs like warfarin and those who are vitamin K deficient should not take vitamin E supplements without close medical supervision because of the increased risk of excessive bleeding (hemorrhage) 251.
Vitamin K Deficiency
Vitamin K deficiency is very rare. People who do not regularly eat a lettuce salad or green leafy vegetables are likely to be vitamin K deficient; national data suggests that only about one in four Americans meets the goal for vitamin K intake from food 184. The current US dietary guidelines for intakes of vitamin K is set at 90 micrograms (mcg)/day for women and 120 mcg/day for men 140, 24. Inadequate intake of vitamin K is unlikely to cause symptoms. Vitamin K deficiency results from extremely inadequate intake, fat malabsorption (eg, due to biliary obstruction, malabsorption disorders, cystic fibrosis, or resection of the small intestine) or use of coumarin anticoagulants. Vitamin K deficiency is particularly common among breastfed infants 252. Vitamin K deficiency is only considered clinically relevant when prothrombin time (PT) increases significantly due to a decrease in the prothrombin activity of blood 24, 32. Therefore, easy bruising and bleeding problems that may be manifested as nosebleed (epistaxis), hematoma (abnormal collection of blood outside of a blood vessel), gastrointestinal bleeding with blood in the stool or tarry black stools (melena), extremely heavy menstrual bleeding (menorrhagia), blood in the urine (hematuria), bleeding gums, and oozing from venipuncture sites are the classic signs of vitamin K deficiency, although these effects occur only in severe cases. Easy bruisability also is observed 253. In infants, vitamin K deficiency may result in life-threatening bleeding within the skull (intracranial hemorrhage) 248. Because vitamin K is required for the carboxylation of osteocalcin in bone, vitamin K deficiency could also reduce bone mineralization and reduce bone strength and increase the risk of getting osteoporosis because the body needs vitamin K for healthy bones 254.
Several studies suggest that low vitamin K levels are related to osteoporosis, pathological fractures and vascular calcifications 20. Supplementing menaquinone-7 (MK-7) at the dose of at least 200 mcg per day might help protecting from vascular calcification, osteoporosis and cancer 86. Moreover, supplementation of 5 mg daily phylloquinone (vitamin K1) in 440 postmenopausal women with osteopenia for 2 years in a randomized, placebo-controlled, double-blind trial caused a > 50% reduction in clinical fractures vs. placebo, although no protection against the age-related decline in bone mineral density was observed 87.
A meta-analysis has shown that in seven Japanese trials reporting fractures, vitamin K2 (menaquinones) administration significantly reduced the risk of hip (77% reduction), vertebral (60% reduction) and all non-vertebral fractures (81% reduction) 88. Vitamin K administration also significantly delayed the progression of coronary artery calcifications and the deterioration of arterial elasticity 89. A lower risk of coronary heart disease and severe aortic calcifications was observed with higher menaquinones intake, but not with phylloquinone intake. This finding suggests that the dietary phylloquinone intake, without menaquinones, may not be sufficient to suppress arterial calcifications 90.
Vitamin K2 (menaquinones) have been shown to play an important role also in cancer. In a small (40 patients) randomized study the administration of menaquinones 45 mg/day reduced the development of hepatocellular carcinoma in patients with liver cirrhosis: the risk ratio for the development of hepatocellular carcinoma in patients given menaquinones was 0.13 91.
Vitamin K2 (menaquinones) have additional properties in certain cell and tissue types, particularly in bone tissue and in the immune system. Much of the available evidence relates specifically to menaquinone-4 (MK-4), which was found to have a role in bone health since the 1990s. Low circulating levels of vitamin K2 (menaquinones) are associated with osteoporotic fractures in the elderly 92 and vitamin K2 (menaquinones) improved bone mineral density in Japanese women 93. In an experimental setting, MK-4 reduced bone losses caused by either estrogen withdrawal or corticosteroid treatment in experimental model on rats 94, 95. Moreover, other in vitro studies showed that menaquinone-4 (MK-4) inhibits the synthesis of prostaglandin E2 (PGE2), a bone reabsorption-inducing agent, in cultured osteoblasts 96 and inhibits the formation of osteoclast-like cells in bone marrow-derived cultures 97. Finally, experimental data suggests a possible role of MK-4 on pancreatic exocrine cells metabolism. Stimulation of pancreatic acinar cells with secretagogues cholecystokinin-8 and secretin induces secretion of MK-4, along with phospholipase and the membrane trafficking protein caveolin-1 98, although a well-defined function of MK-4 in this setting remains unclear.
In the Vitamin K Italian (VIKI) study 99, a comprehensive assessment of vitamin K status was carried out in a cohort of hemodialysis patients and in healthy controls, including most vitamin K subtypes (in particular phylloquinone [vitamin K1], MK-4, MK-5, MK-6, and MK-7), adjusted for triglycerides levels. Vitamin K deficiency was found in 35.4% of hemodialysis patients for MK-7, 23.5% for PK and 14.5% for MK-4. With the limitations of its observational nature, this is the first study to relate vitamin K1 (phylloquinone) and vitamin K2 (menaquinones) deficiency directly both to vertebral fractures and vascular calcification in the dialysis population 99. In particular, vitamin K1 (phylloquinone) deficiency was the strongest predictor of vertebral fractures, while lower MK-4 and MK-7 levels were associated with vascular calcification. The results in hemodialysis patients may point out a possible role of vitamin K deficiency as a cause of bone and vascular disease also in the general population. It can be hypothesized that a diet rich in vitamin K and/or vitamin K supplements might be of help in preventing bone disease and avoiding vascular calcifications, opening interesting perspectives for research in human health.
The frequent use of warfarin enhances the problem of vitamin K deficiency and its role on bone and vascular disease 100. Warfarin may predispose to bone fractures and vascular calcification by different mechanisms: directly, by inhibition of gamma-carboxylation of osteocalcin and other bone matrix proteins; indirectly, because patients treated with warfarin may limit their dietary intake of foods rich in vitamin K. New oral anticoagulant seems to have less influence on bone metabolism, but their long-term effects need more studies 101, 102.
Finally, vitamin K status was found to be inversely and significantly related to individual inflammatory markers and to the inflammatory process in a human population study based on the Framingham Offspring Study cohort 103. This finding is supported by studies on rats demonstrating that animals with vitamin K-deficient diets had an enhanced expression of genes involved in acute inflammatory response compared to those with normal or phylloquinone-supplemented diets and that a supplemented diet suppressed the inflammatory response 104.
Most people in the United States get enough vitamin K from the foods they eat. Also, bacteria in the colon make some vitamin K that the body can absorb. However, certain groups of people may have trouble getting enough vitamin K:
- Newborns who don’t receive an injection of vitamin K at birth
- People with conditions (such as cystic fibrosis, celiac disease, ulcerative colitis, and short bowel syndrome) that decrease the amount of vitamin K their body absorbs
- People who have had bariatric (weight loss) surgery
Certain antibiotics (particularly some cephalosporins and other broad-spectrum antibiotics), salicylates, megadoses of vitamin E, and individuals with significant liver damage or disease increase risk of bleeding in patients with vitamin K deficiency 248. Additionally, individuals with fat malabsorption disorders, including inflammatory bowel disease and cystic fibrosis, may be at increased risk of vitamin K deficiency 254, 255, 256, 257.
Vitamin K deficiency can occur during the first few weeks of infancy due to low placental transfer of phylloquinone, low clotting factor levels, and low vitamin K content of breast milk 32. Clinically significant vitamin K deficiency in adults is very rare and is usually limited to people with malabsorption disorders or those taking drugs that interfere with vitamin K metabolism 82, 32, 20, 258, 259. In healthy people consuming a varied diet, achieving a vitamin K intake low enough to alter standard clinical measures of blood coagulation is almost impossible 24.
Neonates are prone to vitamin K deficiency because of the following:
- The placenta transmits lipids and vitamin K relatively poorly.
- The neonatal liver is immature with respect to prothrombin synthesis.
- Breast milk is low in vitamin K, containing about 2.5 mcg/L (cow’s milk contains 5000 mcg/L).
- The neonatal gut is sterile during the first few days of life.
Treatment consists of vitamin K given orally or, when fat malabsorption is the cause or when risk of bleeding is high, parenterally.
Currently, there is not a consensus on a plasma vitamin K level indicating deficiency or insufficiency. Similarly, it is not clear which vitamer should be considered as reference for determining the vitamin K status 20. Data obtained in healthy subjects and osteoporotic patients supplemented with menaquinone-4 (MK-4) showed a large variability of vitamin K levels. In healthy subjects, levels of MK-4, vitamin K1 (phylloquinone) and MK-7 (reported as ng/mL and mean ± SD) were 0.15 ± 0.17, 1.81 ± 1.10 and 16.27 ± 20.58, respectively, while in osteoporotic patients receiving MK-4, these levels were 46.83 ± 46.41, 0.62 ± 0.25 and 4.18 ± 6.28, respectively 39. The influence of supplementation on menaquinone-4 (MK-4) levels was also observed in another study involving postmenopausal Japanese women 40, in contrast with the low MK-4 bioavailability reported in humans by Sato 41. Other authors measured vitamin K1 (phylloquinone) levels, demonstrating that vitamin K deficiency affects 24% of the general population and 29% of hemodialysis patients 42, 43. Vitamin K deficiency impairs blood coagulation process leading to issues with bleeding. Recent research has linked vitamin K deficiency to issues with osteoporosis and cystic fibrosis 44, 45, 11.
Protein induced by vitamin K absence (PIVKA-II) also known as des-gamma-carboxy prothrombin (DCP), is currently the best test commonly available to determine vitamin K status 260, 257. PIVKA-II or des-gamma-carboxy prothrombin (DCP) is an abnormal form of prothrombin, a clotting factor produced by the liver. In the absence of vitamin K, there is a production of PIVKA-II and is a sensitive marker for vitamin K deficiency status 257. PIVKA-II levels reflect the functional marker of coagulation. PIVKA-II has minimal variability based on other factors such as age that influence vitamin K plasma and serum concentration 261. Increased PIVKA-II levels start to become apparent in individuals consuming less than 60 mcg of vitamin K per day 262. At birth, elevated PIVKA-II levels exist in 10-50% of newborns and 70% of non-supplemented healthy infants on day of life 4 or 5 263.
The monitoring of vitamin K administration or levels is usually through prothrombin time (PT) and INR (international normalized ratio). These values measure the presence of vitamin K-dependent factors, which is especially important to utilize in patients who have warfarin toxicity or vitamin K-related bleeding disorders (coagulopathies).
Vitamin K Deficiency causes
In infants, the low transmission of vitamin K across the placenta, liver prematurity with prothrombin synthesis, lack of vitamin K in breast milk, and the sterile gut in neonates account for vitamin K deficiency 264. Neonatal diseases that cause cholestasis can result in vitamin K deficiency 156. Parents refusal of vitamin K prophylaxis at childbirth can result in bleeding complications 156.
Hereditary combined vitamin K-dependent clotting factor deficiency (VKCFD) is a rare autosomal recessive congenital bleeding disorder that is characterized by decreased levels of the vitamin K-dependent pro- and anticoagulant factors; and vitamin K-dependent proteins involved in calcium homeostasis, bone and cartilage formation 265, 266, 267, 268, 269. The two genes associated with the hereditary combined vitamin K-dependent clotting factor deficiency (VKCFD) are GGCX (gamma-glutamyl carboxylase) and VKORC1 (vitamin K epoxide reductase complex subunit 1) 270, 271. Hereditary combined vitamin K-dependent clotting factor deficiency (VKCFD) can manifest as a spectrum of presentations, with severity ranging from mild to severe 268. The first case of VKCFD was described in 1966, in a female newborn who exhibited significant bleeding from the first week of life 272. The child was born from an uncomplicated pregnancy and had symptoms manifesting as bruising beginning at week 1 of life, with recurrent serosanguinous oozing from the umbilical stump throughout the first months of life 272. The diagnosis of hereditary combined vitamin K-dependent clotting factor deficiency (VKCFD) is extremely rare. Currently, fewer than 30 VKCFD cases worldwide have been reported and affects males and females equally 268, 273, 274, 275. Most patients with VKCFD show partial or complete improvement in factor activity, as well as normalization of prothrombin time (PT) and activated partial thromboplastin time (aPTT) with oral or parenteral vitamin K 276, 277, 278. These patients have an excellent prognosis. In some cases, though, vitamin K is ineffective and there is biochemical evidence that the molecules are not fully carboxylated by vitamin K treatment 279, 280. The response to vitamin K varies based on the route of administration and the individual’s sensitivity to vitamin K 281. A fixed therapeutic regimen has not been identified, and no clear correlation exists between clinical severity and responsiveness to vitamin K 268. Continued daily treatment with high-dose oral vitamin K has been successful in preventing some bleeding complications and is recommended for patients with VKCFD 279, 277. One case report also suggested possible value in administering fresh frozen plasma for VKCFD in pregnancy while another suggested the use of prothrombin complex concentrates, which contain factors II, VII, IX, and X, and proteins C and S 277, 282.
In adults, the causes of vitamin K deficiency include the following 283:
- Chronic illness
- Malnutrition
- Alcoholism
- Multiple abdominal surgeries
- Long-term parenteral nutrition
- Malabsorption syndromes
- Infectious diarrhea
- Cholestatic disease
- Parenchymal liver disease
- Cystic fibrosis (CF)
- Inflammatory bowel disease
- Drugs – Antibiotics (cephalosporin), cholestyramines, warfarin, salicylates, anticonvulsants, and certain sulfa drugs) are some of the common causes of vitamin K deficiency
- Massive transfusion
- Disseminated intravascular coagulation (DIC) – Severe
- Chronic kidney disease/hemodialysis 284
The synthesis of vitamin K-dependent factors are decreased by parenchymal liver diseases, such as cirrhosis secondary to viral hepatitis, alcohol intake, and other infiltrative diseases; hepatic malignancy; amyloidosis; Gaucher disease; and alpha-1 antitrypsin deficiency. Therefore, supplementation with vitamin K is not effective unless a patient has severe bleeding and fresh frozen plasma is administered in addition to correcting the coagulopathy.
Malabsorption syndrome affects vitamin K absorption in the ileum. Celiac sprue, tropical sprue, Crohn disease, ulcerative colitis, Ascaris infection, bacterial overgrowth, chronic pancreatitis, and short bowel syndrome resulting from multiple abdominal surgeries can result in poor absorption of vitamin K (which can be corrected with vitamin K supplementation) 285.
Cystic fibrosis patients who have pancreatic insufficiency, excessive or chronic antibiotic usage, or short bowel due to intestinal resection are at increased risk for vitamin K deficiency due to malabsorption 286.
Biliary diseases, such as common duct obstruction due to stones and strictures, primary biliary cirrhosis, cholangiocarcinoma, and chronic cholestasis, cause maldigestion of fat. The decrease in fat absorption leads to a deficiency of fat-soluble vitamins, such as vitamin K 264. In addition, surgery and T-tube drainage of the bile duct can lead to a vitamin K-deficient state.
Dietary deficiency occurs in people with malnutrition, alcoholics, and patients undergoing long-term parenteral nutrition without vitamin K supplements. A large amount of vitamin E can antagonize vitamin K and prolong the prothrombin time (PT).
Various drugs, such as cholestyramine, bind to bile acids, thus preventing fat-soluble vitamin absorption. Warfarin blocks the effect of vitamin K epoxide reductase and vitamin K reductase, thereby inducing an intracellular deficiency. Cefamandole, cefoperazone, salicylates, hydantoins, rifampin, isoniazid, and barbiturates are some of the common drugs that are associated with vitamin K deficiency, but their mechanism of action in this condition is unknown.
Because 2 main sources of vitamin K exist, neither dietary deficiency nor gut sterilization produces significant bleeding disorder in a healthy person.
Groups at risk of vitamin K deficiency
Vitamin K deficiency can occur in any age group, but it is encountered most often in infancy. In the United States, the prevalence of vitamin K deficiency varies by geographic region 287. In infants, vitamin K deficiency without bleeding may occur in as many as 50% of infants younger than 5 days. The classic hemorrhagic disease occurs in 0.25-1.7% of infants. The prevalence of late hemorrhagic disease in breastfed infants is about 20 cases per 100,000 live births with no prior vitamin K prophylaxis.
Comparing incidences of vitamin K deficiency between different countries is difficult because countries have different criteria to acquire their national incidences. Among countries that share the same methodologies, western European countries have an incidence of late vitamin K deficiency bleeding in infants of approximately 5 cases per 105 live births; the incidence is 11 cases per 105 live births in Japan; and the incidence is 72 cases per 105 live births in Thailand 288.
Vitamin K deficiency is uncommon in healthy adults for a number of reasons: (1) vitamin K is widespread in foods (see Food sources); (2) the vitamin K cycle conserves vitamin K (see Vitamin K oxidation-reduction cycle); and (3) bacteria that normally inhabit the large intestine synthesize menaquinones (vitamin K2), although it is unclear whether significant amounts are absorbed and utilized (see Food sources). Adults at risk for vitamin K deficiency include those taking vitamin K antagonists and individuals with significant liver damage or disease 248. Additionally, individuals with fat malabsorption disorders, including inflammatory bowel disease and cystic fibrosis, may be at increased risk of vitamin K deficiency 254, 255, 256, 257.
The following groups are among those most likely to have inadequate vitamin K status.
Newborns not treated with vitamin K at birth
Worldwide, vitamin K deficiency causes infant morbidity and mortality. Vitamin K deficiency causes hemorrhagic disease of the newborn or vitamin K deficiency bleeding (VKDB) of the newborn, which usually occurs 1 to 7 days postpartum 289. In affected neonates, birth trauma can cause bleeding inside the skull (intracranial hemorrhage). A late form of this disease can occur in infants about 2 to 12 weeks old, typically in infants who are breastfed and are not given vitamin K supplements. Newborn babies who are exclusively breast-fed are at increased risk for vitamin K deficiency because human breast milk is relatively low in vitamin K compared to milk formula. Newborn infants, in general, have low vitamin K status for the following reasons: (1) vitamin K transport across the placental barrier is limited; (2) liver storage of vitamin K is very low; (3) the vitamin K cycle may not be fully functional in newborns, especially premature infants; (4) the vitamin K content of breast milk is low, and immature gut flora 290, 291. If the mother has taken phenytoin anticonvulsant medication to prevent seizures, coumarin anticoagulants, or cephalosporin antibiotics, the risk of hemorrhagic disease is increased due to vitamin K deficiency 289. Because hemorrhagic disease of the newborn or vitamin K deficiency bleeding of the newborn is life threatening and easily prevented, the American Academy of Pediatrics and a number of similar international organizations recommend that an intramuscular dose of 0.5 to 1 milligram (mg) of phylloquinone (vitamin K1) be administered to all newborns shortly after birth 292, 232.
Vitamin K transport across the placenta is poor, increasing the risk of vitamin K deficiency in newborn babies. During the first few weeks of life, vitamin K deficiency can cause vitamin K deficiency bleeding, a condition formerly known as “classic hemorrhagic disease of the newborn.” Vitamin K deficiency bleeding is associated with bleeding in the umbilicus, gastrointestinal tract, skin, nose, or other sites 32, 232, 293. Vitamin K deficiency bleeding (VKDB) of the newborn also known as “early vitamin K deficiency bleeding” when it occurs in the first week of life. “Late vitamin K deficiency bleeding” occurs at ages 2–12 weeks, especially in exclusively breastfed infants due to the low vitamin K content of breast milk or in infants with malabsorption problems (such as cholestatic jaundice or cystic fibrosis) 32. Vitamin K deficiency bleeding, especially late vitamin K deficiency bleeding, can also be manifested as sudden intracranial bleeding, which has a high mortality rate 32, 293.
People with malabsorption disorders
People with malabsorption syndromes and other gastrointestinal disorders, such as cystic fibrosis, celiac disease, ulcerative colitis, and short bowel syndrome, might not absorb vitamin K properly 1, 294. Vitamin K status can also be low in patients who have undergone weight loss surgery (bariatric surgery), although clinical signs may not be present 295. These individuals might need monitoring of vitamin K status and, in some cases, vitamin K supplementation.
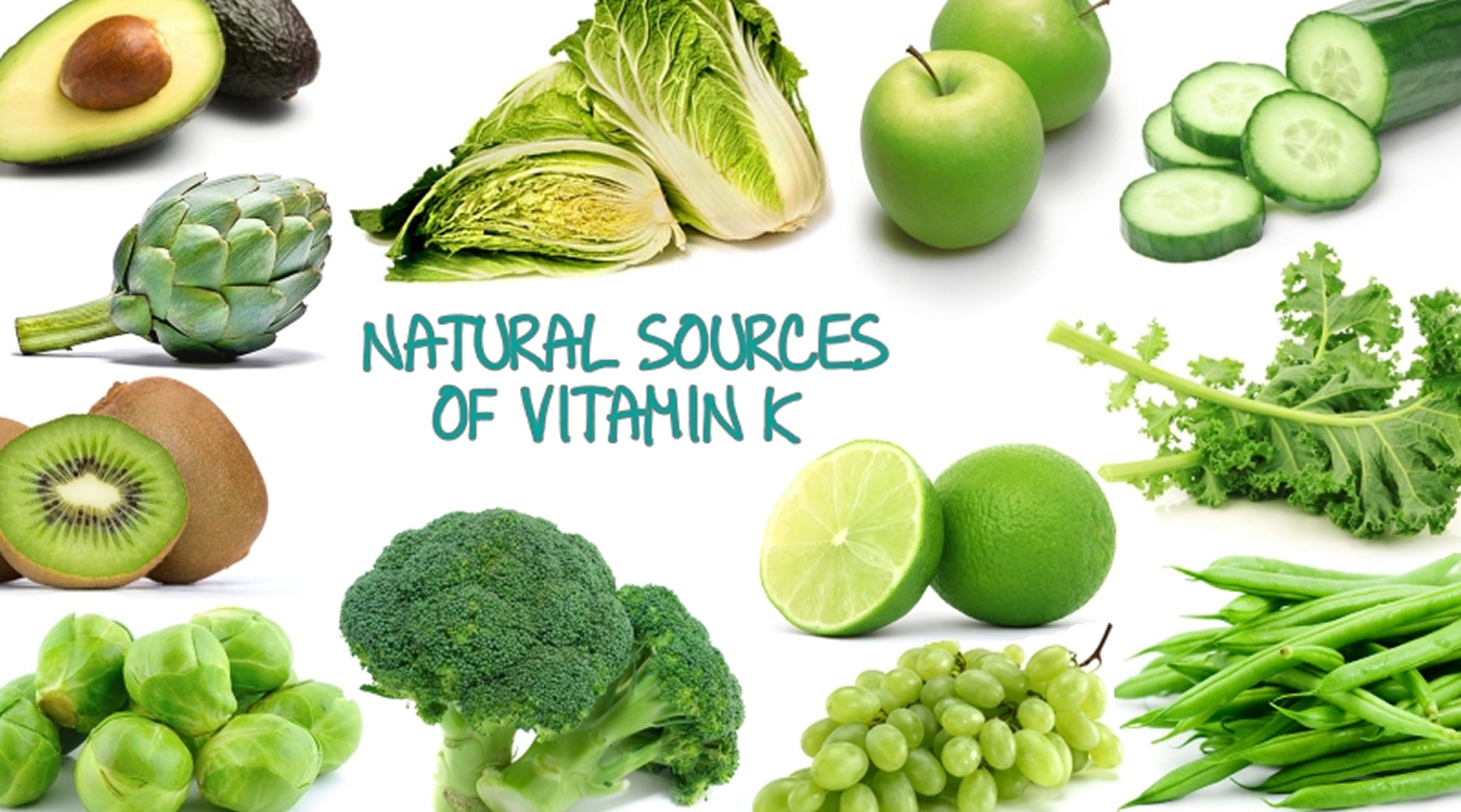
Vitamin K deficiency signs and symptoms
The clinical manifestations of vitamin K deficiency are evident only if hypoprothrombinemia is present. Bleeding is the major symptom, especially in response to minor or trivial trauma. Any site can be involved, so manifestations can include mucosal and subcutaneous bleeding, such as nosebleed (epistaxis), hematoma (abnormal collection of blood outside of a blood vessel), gastrointestinal bleeding with blood in the stool or tarry black stools (melena), extremely heavy menstrual bleeding (menorrhagia), blood in the urine (hematuria), bleeding gums, and oozing from venipuncture sites. Easy bruisability also is observed 253. In infants, vitamin K deficiency may result in life-threatening bleeding within the skull (intracranial hemorrhage) 248. Vitamin K deficiency bleeding (VKDB) in newborns can separate into three categories based on the timing of the presentation. Early vitamin K deficiency bleeding (VKDB) in newborns presents within 24 hours after birth, classic VKDB presents within the first week, and late VKDB presents between one to twelve weeks of life 263.
In vitamin K deficiency bleeding (VKDB), the neonate will present with bleeding. The first reported classic vitamin K deficiency bleeding (VKDB) in newborns was in 1894 as a bleeding disorder that occurred on day 2 or 3 of life 296. In combination with sepsis-induced bleeding, the incidence was 600/100,000 infants with a 62% fatality rate 296. Late vitamin K deficiency bleeding (VKDB) in newborns occurs in 4.4 to 72 infants per 100,000 births with an increased risk in exclusively breastfed infants and the highest incidence occurring in Asian populations 296. Early VKDB has been associated with mothers on anticonvulsants or other vitamin K interfering substances, and incidence without vitamin K supplementation has been reported as high as 12% 296. The mortality rate for late vitamin K deficiency bleeding (VKDB) in newborns is 20-50%. Late vitamin K deficiency bleeding (VKDB) in newborns also has a significant neurologic morbidity rate. Without Vitamin K supplementation, the current day incidence of classic VKDB is estimated to be 0.25-1.7% 263, 297.
Early vitamin K deficiency bleeding (VKDB) in newborns often presents with intracranial, intrathoracic, intra-abdominal and other severe bleeding conditions. Early VKDB is also often associated with maternal drugs that inhibit vitamin K metabolism. Classis VKDB typically occurs with less severe bleeding such as that of the umbilicus, gastrointestinal tract, and post-circumcision. Late VKDB often presents with severe intracranial bleed. All forms of VKDB have a high incidence of the refusal of vitamin K prophylaxis. Late VKDB has a higher association with exclusively breastfed infants due to the lower dietary intake of vitamin K found in human milk versus formula 289. Warning bleeds or bruising should always prompt further investigation by laboratory testing.
Hereditary combined vitamin K-dependent clotting factor deficiency (VKCFD) presents in the newborn period in severe cases similar to vitamin K deficiency bleeding (VKDB) in newborns but can present later in life in milder cases. Symptoms of VKCFD can vary and correlate with coagulation factor levels 298. Affected newborns can be symptomatic at birth with spontaneous intracranial hemorrhage or umbilical stump bleeding 275, 299, 300. Alternatively, they can be asymptomatic at first and present later in childhood with spontaneous hemarthrosis or soft-tissue or gastrointestinal bleeds 301. Older individuals can have easy bruising or post-surgical bleeding 276, 302. Other signs and symptoms in more severely affected children with the hereditary combined vitamin K-dependent clotting factor deficiency (VKCFD) GGCX mutations, include skeletal abnormalities such as bone hypoplasia, conductive hearing loss, and mental retardation 278, 303, 266, 268.
Because vitamin K is required for the carboxylation of osteocalcin in bone, vitamin K deficiency could also reduce bone mineralization and reduce bone strength and increase the risk of getting osteoporosis because the body needs vitamin K for healthy bones 254.
Physical examination
Ecchymosis, petechiae, hematomas, and oozing of blood at surgical or puncture sites are observed. In infants, some birth defects, such as underdevelopment of the face, nose, bones, and fingers, are linked to a vitamin K-deficient state. Infants may present with nontraumatic intracranial bleeding with signs such as vomiting, poor intake, anemia, seizures, and bleeding in mucosal sites.
Vitamin K deficiency complications
The characteristics of vitamin K deficiency vary according to the age of onset. In infants, vitamin K deficiency causes hemorrhagic disease of newborn, resulting in intracranial and retroperitoneal bleeding, which can occur at 1-7 days postpartum. Late hemorrhagic disease of newborn can occur as late as 3 months postpartum 304.
Because vitamin K is involved in gamma carboxylation of osteocalcin, which is important in bone synthesis, osteoporosis is associated with vitamin K deficiency 305. Osteocalcin is important in the remodeling and mineralization of bone. Increased fracture rates and cardiac disease may also be a complication. However, more research is required 296.
Vitamin K deficiency diagnosis
Measurements of serum prothrombin time (PT) tend to be elevated and activated partial thromboplastin time (aPTT) is usually normal 285, 306). Both PT and aPTT can be elevated in more severe vitamin K deficiency states.
Protein induced by vitamin K absence (PIVKA-II) also known as des-gamma-carboxy prothrombin (DCP), is currently the best test commonly available to determine vitamin K status 260, 257. PIVKA-II or des-gamma-carboxy prothrombin (DCP) is an abnormal form of prothrombin, a clotting factor produced by the liver. In the absence of vitamin K, there is a production of PIVKA-II and is a sensitive marker for vitamin K deficiency status 257. PIVKA-II levels reflect the functional marker of coagulation. PIVKA-II has minimal variability based on other factors such as age that influence vitamin K plasma and serum concentration 261. Increased PIVKA-II levels start to become apparent in individuals consuming less than 60 mcg of vitamin K per day 262. At birth, elevated PIVKA-II levels exist in 10-50% of newborns and 70% of non-supplemented healthy infants on day of life 4 or 5 263.
Direct measurement of vitamin K plasma levels shows highly variable data influenced by the analytical method, nutritional and metabolic factors, and interference of lipid content. Liquid chromatography-tandem mass spectrometry is useful for determining vitamin K subtypes and concentration levels but is not readily available 20.
The plasma level of vitamin K, serum phylloquinone, (0.2-1.0 ng/mL) can be measured; however, the level of vitamin K depends on the oral intake of vitamin K, which can vary. However, a low serum phylloquinone (< 0.15 mcg/L) suggests low tissue body stores 288.
A clearly prolonged PT (international normalized ratio (INR) > 3.5) along with normal fibrinogen concentration and platelet count is highly suggestive of vitamin K deficiency-related bleeding. Confirmation of the diagnosis requires measurement of the specific vitamin K-dependent factors (II, VII, IX, X) whose levels are rapidly corrected by the parenteral administration of 1 mg vitamin K 307.
Diagnostic criteria for vitamin K deficiency bleeding (VKDB) in newborns includes a prothrombin time (PT) greater than or equal to 4 times the normal value and one of the following 296:
- Normal or increased platelet count with normal fibrinogen and absent degradation products
- Prothrombin time (PT) normalization within 30 minutes after IV vitamin K administration
- Increased levels of PIVKA-II 263.
When hereditary combined vitamin K-dependent clotting factor deficiency (VKCFD) is suspected as the cause, a research laboratory can be employed to perform genotyping of gamma-glutamyl carboxylase (GGCX) and VKORC1 (vitamin K epoxide reductase complex subunit 1) 268.
Vitamin K deficiency treatment
The medical therapy for vitamin K deficiency depends on the severity of the associated bleeding and the underlying disease state. The most effective approach to correcting the deficiency also depends on the nature of the bleeding and the risk of inducing a local hematoma at the vitamin K injection site. In life-threatening bleeds, fresh frozen plasma should be administered prior to vitamin K.
Phytonadione (vitamin K1)
Whenever possible, phytonadione (vitamin K1) should be given orally or subcutaneously 252. The usual adult dose is 1 to 20 mg, depending on the severity. Rarely, even when phytonadione is correctly diluted and given slowly, IV replacement can result in anaphylaxis or anaphylactoid reactions. The absorption with the oral form is variable because it requires bile salts in the ileum for absorption. Oral phytonadione (vitamin K1) is used in the setting of asymptomatic vitamin K deficiency. International normalized ratio (INR) usually decreases within 6 to 12 hours. The dose may be repeated in 6 to 8 hours if INR has not decreased satisfactorily. If the PT does not normalize after vitamin K supplementation, then consideration should be made for the presence of liver disease or disseminated intravascular coagulation (DIC).
Phytonadione 1 to 10 mg orally is indicated for nonemergency correction of a prolonged international normalized ratio (INR) in patients taking anticoagulants. Correction usually occurs within 6 to 8 hours. When only partial correction of INR is desirable (eg, when INR should remain slightly elevated because of a prosthetic heart valve), lower doses (eg, 1 to 2.5 mg) of phytonadione can be given.
In infants, bleeding due to vitamin K deficiency can be corrected by giving phytonadione 1 mg sc or IM once. The dose is repeated if INR remains elevated. Higher doses may be necessary if the mother has been taking oral anticoagulants.
In urgent situations, 10-20 mg of injectable phytonadione (vitamin K-1) can be dissolved in a 5% dextrose or 0.9% normal saline to be administered intravenously at a rate not to exceed 1 mg/mL to prevent a hypersensitive or anaphylactic reaction. When giving vitamin K in the intravenous form, the patient needs to be monitored closely, because cardiopulmonary arrest and/or shock can occur in rare cases. The parenteral administration of vitamin K-1 corrects vitamin K deficiency in 12-24 hours.
There is currently no consensus on dosing for chronic supplementation for patients with cystic fibrosis. However, because of limited stores of vitamin K and its fast turnover in the body, daily supplementation is recommended. Dosages for all ages range from 0.3 to 1 mg/day 286.
Prophylaxis in newborns
1 mg of phytonadione (vitamin K1) by intramuscular injection within 1 hour of birth. Alternatively, 2 mg of vitamin K1 orally at birth, at 4-6 days, and at 4-6 weeks. Another alternative oral administration is 2 mg Vitamin K1 at birth and a subsequent weekly dose of 1 mg for three months. Intramuscular injection is preferable for efficacy 263.
Vitamin K deficiency bleeding (VKDB) in newborns
1 to 2 mg phytonadione (vitamin K1) by slow intravenous or subcutaneous infusion. Severe bleeding may require fresh frozen plasma at a dose of 10-15 mL/kg 289.
Hereditary combined vitamin K-dependent clotting factor deficiency (VKCFD)
10 mg vitamin K1 2-3 times per week by an oral dose by intravenous infusion. Fresh frozen plasma may be required during surgery or in cases of severe bleeding at a dose of 15-20 mL/kg of body weight given intravenously, to be repeated until clinical efficacy and amelioration of clotting times 308. The requirement of multiple administrations to reach efficient clotting may be complicated by circulatory overload 308. Prothrombin Complex Concentrates (products containing factors II, VII, IX and X as well as protein C and protein S at variable concentrations) and recombinant Factor VII (Eptacog alfa (activated)) may also have utility during surgery or severe bleeding 268, 309.
Vitamin K deficiency due to malabsorption
Dependent on the disease. Malabsorption requires daily administration of high doses of oral vitamin K1 0.3 to 15 mg/day. If oral dosing is ineffective, consideration should be for parenteral vitamin K1 310.
Vitamin K deficiency in adults due to poor nutrition
At least 120 and 90 mcg/day vitamin K1 for men and women respectively, by diet or oral supplementation to meet the National Academy of Science Food and Nutrition Board recommended intake.
Vitamin K deficiency due to chronic conditions
As more research becomes available, a larger dosage of oral vitamin K1 and K2 may be beneficial. No present guidelines are available 296.
Vitamin K deficiency prognosis
Adults with vitamin K deficiency due to nutritional deficiencies in adults are difficult to evaluate given confounding factors such as overall quality of diet and differences in metabolism due to comorbid conditions or genetics but are considered to have an excellent prognosis if the condition is recognized early and treated appropriately. No mortalities from vitamin K deficiency have been reported. However, severe bleeding can occur if the deficiency is left untreated. Morbidity correlates with the severity of vitamin K deficiency. The risk of developing vitamin K deficiency bleeding is 81 times greater in infants who do not receive a prophylactic vitamin K injection 311.
Prophylaxis with 1 mg of phytonadione (vitamin K1) by intramuscular injection within 1 hour of birth reduces the incidence of vitamin K deficiency bleeding (VKDB) in newborns significantly. Late vitamin K deficiency bleeding (VKDB) in newborns has the worse prognosis, with 50% of cases presenting with intracranial hemorrhage, which is associated with high mortality and morbidity 289.
In hereditary combined vitamin K-dependent clotting factor deficiency (VKCFD), with vitamin K supplementation, there is a good prognosis with a low impact on quality of life, with permanent disabling consequences only resulting in severely affected patients who have been diagnosed after critical events such as intracranial haemorrhage 268.
- Suttie JW. Vitamin K. In: Coates PM, Betz JM, Blackman MR, et al., eds. Encyclopedia of Dietary Supplements. 2nd ed. London and New York: Informa Healthcare; 2010:851-60.[↩][↩][↩][↩][↩][↩][↩][↩][↩][↩][↩][↩][↩][↩]
- Hirota, Yoshihisa & Suhara, Yoshitomo. (2019). New Aspects of Vitamin K Research with Synthetic Ligands: Transcriptional Activity via SXR and Neural Differentiation Activity. International Journal of Molecular Sciences. 2019, 20(12), 3006; https://doi.org/10.3390/ijms20123006[↩][↩][↩][↩][↩]
- Booth S. L. (2012). Vitamin K: food composition and dietary intakes. Food & nutrition research, 56, 10.3402/fnr.v56i0.5505. https://doi.org/10.3402/fnr.v56i0.5505[↩][↩][↩][↩][↩][↩][↩][↩][↩][↩][↩][↩][↩][↩][↩][↩][↩][↩]
- Booth SL, Tucker KL, Chen H, Hannan MT, Gagnon DR, Cupples LA, Wilson PW, Ordovas J, Schaefer EJ, Dawson-Hughes B, Kiel DP. Dietary vitamin K intakes are associated with hip fracture but not with bone mineral density in elderly men and women. Am J Clin Nutr. 2000 May;71(5):1201-8. doi: 10.1093/ajcn/71.5.1201[↩][↩]
- Thane CW, Paul AA, Bates CJ, Bolton-Smith C, Prentice A, Shearer MJ. Intake and sources of phylloquinone (vitamin K1): variation with socio-demographic and lifestyle factors in a national sample of British elderly people. Br J Nutr. 2002 Jun;87(6):605-13. doi: 10.1079/BJNBJN2002583[↩][↩][↩]
- Ferland G. Vitamin K. In: Erdman JW, Macdonald IA, Zeisel SH, eds. Present Knowledge in Nutrition. 10th ed. Washington, DC: Wiley-Blackwell; 2012:230-47.[↩][↩][↩][↩][↩][↩][↩][↩][↩][↩]
- National Institute of Health, Office of Dietary Supplements. Vitamin K. https://ods.od.nih.gov/factsheets/VitaminK-HealthProfessional/[↩][↩]
- Gross J, Cho WK, Lezhneva L, Falk J, Krupinska K, Shinozaki K, Seki M, Herrmann RG, Meurer J. A plant locus essential for phylloquinone (vitamin K1) biosynthesis originated from a fusion of four eubacterial genes. J Biol Chem. 2006 Jun 23;281(25):17189-17196. doi: 10.1074/jbc.M601754200[↩]
- Vitamin K. https://lpi.oregonstate.edu/mic/vitamins/vitamin-K[↩][↩][↩][↩][↩][↩][↩][↩]
- Harshman SG, Finnan EG, Barger KJ, Bailey RL, Haytowitz DB, Gilhooly CH, Booth SL. Vegetables and Mixed Dishes Are Top Contributors to Phylloquinone Intake in US Adults: Data from the 2011-2012 NHANES. J Nutr. 2017 Jul;147(7):1308-1313. doi: 10.3945/jn.117.248179[↩]
- Booth SL. Vitamin K: food composition and dietary intakes. Food Nutr Res. 2012;56. doi: 10.3402/fnr.v56i0.5505[↩][↩][↩][↩][↩][↩][↩][↩][↩][↩][↩]
- Holmes MV, Hunt BJ, Shearer MJ. The role of dietary vitamin K in the management of oral vitamin K antagonists. Blood Rev. 2012 Jan;26(1):1-14. doi: 10.1016/j.blre.2011.07.002[↩][↩][↩]
- McKeown NM, Jacques PF, Gundberg CM, Peterson JW, Tucker KL, Kiel DP, Wilson PW, Booth SL. Dietary and nondietary determinants of vitamin K biochemical measures in men and women. J Nutr. 2002 Jun;132(6):1329-34. doi: 10.1093/jn/132.6.1329[↩]
- Piironen V, Koivu T, Tammisalo O, Mattila P. Determination of phylloquinone in oils, margarines and butter by high-performance liquid chromatography with electrochemical detection. Food Chem. 1997;59:473–80.[↩]
- Peterson JW, Muzzey KL, Haytowitz D, Exler J, Lemar L, Booth SL. Phylloquinone (vitamin K-1) and dihydrophylloquinone content of fats and oils. JAOCS. 2002;79:641–46.[↩]
- Elder SJ, Haytowitz DB, Howe J, Peterson JW, Booth SL. Vitamin k contents of meat, dairy, and fast food in the u.s. Diet. J Agric Food Chem. 2006 Jan 25;54(2):463-7. doi: 10.1021/jf052400h[↩][↩][↩][↩][↩][↩][↩][↩][↩]
- Conly JM, Stein K, Worobetz L, Rutledge-Harding S. The contribution of vitamin K2 (menaquinones) produced by the intestinal microflora to human nutritional requirements for vitamin K. Am J Gastroenterol. 1994 Jun;89(6):915-23.[↩][↩][↩]
- Orimo H, Nakamura T, Hosoi T, Iki M, Uenishi K, Endo N, Ohta H, Shiraki M, Sugimoto T, Suzuki T, Soen S, Nishizawa Y, Hagino H, Fukunaga M, Fujiwara S. Japanese 2011 guidelines for prevention and treatment of osteoporosis–executive summary. Arch Osteoporos. 2012;7(1):3-20. doi: 10.1007/s11657-012-0109-9[↩][↩][↩][↩][↩]
- Rønn SH, Harsløf T, Oei L, Pedersen SB, Langdahl BL. The effect of vitamin MK-7 on bone mineral density and microarchitecture in postmenopausal women with osteopenia, a 3-year randomized, placebo-controlled clinical trial. Osteoporos Int. 2021 Jan;32(1):185-191. doi: 10.1007/s00198-020-05638-z[↩][↩]
- Fusaro M, Gallieni M, Rizzo MA, Stucchi A, Delanaye P, Cavalier E, Moysés RMA, Jorgetti V, Iervasi G, Giannini S, Fabris F, Aghi A, Sella S, Galli F, Viola V, Plebani M. Vitamin K plasma levels determination in human health. Clin Chem Lab Med. 2017 May 1;55(6):789-799. doi: 10.1515/cclm-2016-0783[↩][↩][↩][↩][↩][↩][↩][↩][↩][↩][↩][↩]
- Shearer, M.J. Vitamin K. Lancet 1995, 345, 229–234.[↩]
- Suttie, J.W. The importance of menaquinones in human nutrition. Annu. Rev. Nutr. 1995, 15, 399–417.[↩]
- Booth SL. Roles for vitamin K beyond coagulation. Annu Rev Nutr. 2009;29:89-110. doi: 10.1146/annurev-nutr-080508-141217[↩][↩]
- Institute of Medicine (US) Panel on Micronutrients. Dietary Reference Intakes for Vitamin A, Vitamin K, Arsenic, Boron, Chromium, Copper, Iodine, Iron, Manganese, Molybdenum, Nickel, Silicon, Vanadium, and Zinc. Washington (DC): National Academies Press (US); 2001. Available from: https://www.ncbi.nlm.nih.gov/books/NBK222310[↩][↩][↩][↩][↩][↩][↩][↩][↩][↩][↩][↩][↩]
- Imbrescia K, Moszczynski Z. Vitamin K. [Updated 2023 Feb 13]. In: StatPearls [Internet]. Treasure Island (FL): StatPearls Publishing; 2023 Jan-. Available from: https://www.ncbi.nlm.nih.gov/books/NBK551578[↩][↩][↩][↩]
- Davidson RT, Foley AL, Engelke JA, Suttie JW. Conversion of dietary phylloquinone to tissue menaquinone-4 in rats is not dependent on gut bacteria. J Nutr. 1998 Feb;128(2):220-3. doi: 10.1093/jn/128.2.220[↩][↩][↩][↩]
- Thijssen HH, Vervoort LM, Schurgers LJ, Shearer MJ. Menadione is a metabolite of oral vitamin K. Br J Nutr. 2006 Feb;95(2):260-6. doi: 10.1079/bjn20051630[↩][↩][↩][↩]
- Walther, B., Karl, J. P., Booth, S. L., & Boyaval, P. (2013). Menaquinones, bacteria, and the food supply: the relevance of dairy and fermented food products to vitamin K requirements. Advances in nutrition (Bethesda, Md.), 4(4), 463–473. https://doi.org/10.3945/an.113.003855[↩][↩][↩][↩][↩][↩][↩][↩][↩][↩]
- Okano T, Shimomura Y, Yamane M, Suhara Y, Kamao M, Sugiura M, Nakagawa K. Conversion of phylloquinone (Vitamin K1) into menaquinone-4 (Vitamin K2) in mice: two possible routes for menaquinone-4 accumulation in cerebra of mice. J Biol Chem. 2008 Apr 25;283(17):11270-9. doi: 10.1074/jbc.M702971200[↩][↩]
- Wells HF, Buzby JC. Dietary assessment of major trends in U.S. food consumption, 1970–2005. Economic Information Bulletin No (EIB-33) p 27, March 2008.[↩]
- Gast GC, de Roos NM, Sluijs I, Bots ML, Beulens JW, Geleijnse JM, Witteman JC, Grobbee DE, Peeters PH, van der Schouw YT. A high menaquinone intake reduces the incidence of coronary heart disease. Nutr Metab Cardiovasc Dis. 2009 Sep;19(7):504-10. doi: 10.1016/j.numecd.2008.10.004[↩][↩][↩]
- Suttie JW. Vitamin K. In: Ross AC, Caballero B, Cousins RJ, Tucker KL, Ziegler TR, eds. Modern Nutrition in Health and Disease. 11th ed. Baltimore, MD: Lippincott Williams & Wilkins; 2014:305-16.[↩][↩][↩][↩][↩][↩][↩][↩][↩][↩][↩][↩][↩][↩]
- Conversion of dietary phylloquinone to tissue menaquinone-4 in rats is not dependent on gut bacteria. Davidson RT, Foley AL, Engelke JA, Suttie JW. J Nutr. 1998 Feb; 128(2):220-3. https://www.ncbi.nlm.nih.gov/pubmed/9446847/[↩][↩]
- Menadione is a metabolite of oral vitamin K. Thijssen HH, Vervoort LM, Schurgers LJ, Shearer MJ. Br J Nutr. 2006 Feb; 95(2):260-6. https://www.ncbi.nlm.nih.gov/pubmed/16469140/[↩][↩]
- Vitamin K contents of meat, dairy, and fast food in the U.S. Diet. Elder SJ, Haytowitz DB, Howe J, Peterson JW, Booth SL. J Agric Food Chem. 2006 Jan 25; 54(2):463-7. https://www.ncbi.nlm.nih.gov/pubmed/16417305/[↩][↩]
- Schurgers LJ, Vermeer C. Determination of phylloquinone and menaquinones in food. Effect of food matrix on circulating vitamin K concentrations. Haemostasis. 2000;30:298–307. https://www.ncbi.nlm.nih.gov/pubmed/11356998[↩][↩][↩][↩][↩]
- Shearer, M. J., Fu, X., & Booth, S. L. (2012). Vitamin K nutrition, metabolism, and requirements: current concepts and future research. Advances in nutrition (Bethesda, Md.), 3(2), 182–195. https://doi.org/10.3945/an.111.001800[↩][↩]
- Shearer MJ, Newman P. Metabolism and cell biology of vitamin K. Thromb Haemost. 2008 Oct;100(4):530-47.[↩][↩][↩][↩][↩]
- Olson RE. The function and metabolism of vitamin K. Annu Rev Nutr 1984;4:281–337.[↩][↩]
- Koitaya N, Ezaki J, Nishimuta M, Yamauchi J, Hashizume E, Morishita K, et al. Effect of low dose vitamin K2 (MK-4) supplementation on bio-indices in postmenopausal Japanese women. J Nutr Sci Vitaminol (Tokyo) 2009;55:15–21.[↩][↩]
- Sato T, Schurgers LJ, Uenishi K. Comparison of menaquinone-4 and menaquinone-7 bioavailability in healthy women. Nutr J 2012;11:93.[↩][↩]
- Neogi T, Booth SL, Zhang YQ, Jacques PF, Terkeltaub R, Aliabadi P, et al. Low vitamin K status is associated with osteoarthritis in the hand and knee. Arthritis Rheum 2006;54:1255–61.[↩][↩]
- Pilkey RM, Morton AR, Boffa MB, Noordhof C, Day AG, Su Y, et al. Subclinical vitamin K deficiency in hemodialysis patients. Am J Kidney Dis 2007;49:432–9.[↩][↩]
- Tie JK, Stafford DW. Structural and functional insights into enzymes of the vitamin K cycle. J Thromb Haemost. 2016 Feb;14(2):236-47. doi: 10.1111/jth.13217[↩][↩][↩]
- Tanaka N, Arima K, Nishimura T, Tomita Y, Mizukami S, Okabe T, Abe Y, Kawashiri SY, Uchiyama M, Honda Y, Tsujimoto R, Kanagae M, Osaki M, Aoyagi K. Vitamin K deficiency, evaluated with higher serum ucOC, was correlated with poor bone status in women. J Physiol Anthropol. 2020 Apr 10;39(1):9. doi: 10.1186/s40101-020-00221-1[↩][↩]
- Gundberg CM, Lian JB, Booth SL. Vitamin K-dependent carboxylation of osteocalcin: friend or foe? Adv Nutr. 2012 Mar 1;3(2):149-57. doi: 10.3945/an.112.001834[↩]
- Szulc P, Chapuy MC, Meunier PJ, Delmas PD. Serum undercarboxylated osteocalcin is a marker of the risk of hip fracture in elderly women. J Clin Invest. 1993 Apr;91(4):1769-74. doi: 10.1172/JCI116387[↩]
- Vergnaud P, Garnero P, Meunier PJ, Bréart G, Kamihagi K, Delmas PD. Undercarboxylated osteocalcin measured with a specific immunoassay predicts hip fracture in elderly women: the EPIDOS Study. J Clin Endocrinol Metab. 1997 Mar;82(3):719-24. doi: 10.1210/jcem.82.3.3805[↩]
- Shearer MJ. The roles of vitamins D and K in bone health and osteoporosis prevention. Proc Nutr Soc. 1997 Nov;56(3):915-37. doi: 10.1079/pns19970099[↩]
- O’Connor E, Mølgaard C, Michaelsen KF, Jakobsen J, Cashman KD. Vitamin D-vitamin K interaction: effect of vitamin D supplementation on serum percentage undercarboxylated osteocalcin, a sensitive measure of vitamin K status, in Danish girls. Br J Nutr. 2010 Oct;104(8):1091-5. doi: 10.1017/S0007114510001935[↩][↩]
- Kanellakis S, Moschonis G, Tenta R, Schaafsma A, van den Heuvel EG, Papaioannou N, Lyritis G, Manios Y. Changes in parameters of bone metabolism in postmenopausal women following a 12-month intervention period using dairy products enriched with calcium, vitamin D, and phylloquinone (vitamin K(1[↩][↩]
- Bolton-Smith C, McMurdo ME, Paterson CR, Mole PA, Harvey JM, Fenton ST, Prynne CJ, Mishra GD, Shearer MJ. Two-year randomized controlled trial of vitamin K1 (phylloquinone) and vitamin D3 plus calcium on the bone health of older women. J Bone Miner Res. 2007 Apr;22(4):509-19. doi: 10.1359/jbmr.070116[↩]
- Booth SL, Suttie JW. Dietary intake and adequacy of vitamin K. J Nutr. 1998 May;128(5):785-8. doi: 10.1093/jn/128.5.785[↩]
- Garber AK, Binkley NC, Krueger DC, Suttie JW. Comparison of phylloquinone bioavailability from food sources or a supplement in human subjects. J Nutr. 1999 Jun;129(6):1201-3. doi: 10.1093/jn/129.6.1201[↩]
- Booth SL, Lichtenstein AH, Dallal GE. Phylloquinone absorption from phylloquinone-fortified oil is greater than from a vegetable in younger and older men and women. J Nutr. 2002 Sep;132(9):2609-12. doi: 10.1093/jn/132.9.2609[↩]
- Davidson K, Booth S, Dolnikowski G, Sadowski J. Conversion of vitamin K-1 to 2′,3′-dihydrovitamin K1 during the hydrogenation of vegetable oils. J Agric Food Chem. 1996;44:980–83.[↩][↩]
- Booth SL, Lichtenstein AH, O’Brien-Morse M, McKeown NM, Wood RJ, Saltzman E, Gundberg CM. Effects of a hydrogenated form of vitamin K on bone formation and resorption. Am J Clin Nutr. 2001 Dec;74(6):783-90. doi: 10.1093/ajcn/74.6.783[↩]
- Troy LM, Jacques PF, Hannan MT, Kiel DP, Lichtenstein AH, Kennedy ET, Booth SL. Dihydrophylloquinone intake is associated with low bone mineral density in men and women. Am J Clin Nutr. 2007 Aug;86(2):504-8. doi: 10.1093/ajcn/86.2.504[↩]
- Schurgers LJ, Vermeer C. Differential lipoprotein transport pathways of K-vitamins in healthy subjects. Biochim Biophys Acta. 2002 Feb 15;1570(1):27-32. doi: 10.1016/s0304-4165(02)00147-2[↩]
- Thijssen HH, Drittij-Reijnders MJ. Vitamin K status in human tissues: tissue-specific accumulation of phylloquinone and menaquinone-4. Br J Nutr. 1996 Jan;75(1):121-7. doi: 10.1079/bjn19960115[↩]
- Conly JM, Stein K. Quantitative and qualitative measurements of K vitamins in human intestinal contents. Am J Gastroenterol. 1992 Mar;87(3):311-6.[↩]
- Conly JM, Stein K. The production of menaquinones (vitamin K2) by intestinal bacteria and their role in maintaining coagulation homeostasis. Prog Food Nutr Sci. 1992 Oct-Dec;16(4):307-43.[↩]
- Suttie JW. The importance of menaquinones in human nutrition. Annu Rev Nutr. 1995;15:399-417. doi: 10.1146/annurev.nu.15.070195.002151[↩][↩]
- Imbrescia K, Moszczynski Z. Vitamin K. [Updated 2021 Jul 13]. In: StatPearls [Internet]. Treasure Island (FL): StatPearls Publishing; 2022 Jan-. Available from: https://www.ncbi.nlm.nih.gov/books/NBK551578[↩][↩][↩]
- Tie, J. K., & Stafford, D. W. (2016). Structural and functional insights into enzymes of the vitamin K cycle. Journal of thrombosis and haemostasis : JTH, 14(2), 236–247. https://doi.org/10.1111/jth.13217[↩][↩]
- Oldenburg J, Marinova M, Müller-Reible C, Watzka M. The vitamin K cycle. Vitam Horm 2008;78:35–62.[↩][↩]
- Fusaro M, Crepaldi G, Maggi S, Galli F, D’Angelo A, Calò L, et al. Vitamin K, bone fractures, and vascular calcifications in chronic kidney disease: an important but poorly studied relationship. J Endocrinol Invest 2011;34:317–23.[↩]
- Nelsestuen GL, Zytkovicz TH, Howard JB. The mode of action of vitamin K. Identification of gamma-carboxyglutamic acid as a component of prothrombin. J Biol Chem. 1974 Oct 10;249(19):6347-50.[↩]
- Vermeer C. (1990). Gamma-carboxyglutamate-containing proteins and the vitamin K-dependent carboxylase. The Biochemical journal, 266(3), 625–636. https://www.ncbi.nlm.nih.gov/pmc/articles/PMC1131186/pdf/biochemj00187-0011.pdf[↩]
- Nelsestuen GL. Enhancement of vitamin-K-dependent protein function by modification of the gamma-carboxyglutamic acid domain: studies of protein C and factor VII. Trends Cardiovasc Med. 1999 Aug;9(6):162-7. doi: 10.1016/s1050-1738(99)00024-9[↩]
- Ducy P, Desbois C, Boyce B, Pinero G, Story B, Dunstan C, Smith E, Bonadio J, Goldstein S, Gundberg C, Bradley A, Karsenty G. Increased bone formation in osteocalcin-deficient mice. Nature. 1996 Aug 1;382(6590):448-52. doi: 10.1038/382448a0[↩][↩]
- Ferron M, Lacombe J. Regulation of energy metabolism by the skeleton: osteocalcin and beyond. Arch Biochem Biophys. 2014 Nov 1;561:137-46. doi: 10.1016/j.abb.2014.05.022[↩][↩]
- Booth, S. L., Centi, A., Smith, S. R., & Gundberg, C. (2013). The role of osteocalcin in human glucose metabolism: marker or mediator?. Nature reviews. Endocrinology, 9(1), 43–55. https://doi.org/10.1038/nrendo.2012.201[↩][↩]
- Schurgers LJ, Cranenburg EC, Vermeer C. Matrix Gla-protein: the calcification inhibitor in need of vitamin K. Thromb Haemost. 2008 Oct;100(4):593-603.[↩][↩]
- Willems BA, Vermeer C, Reutelingsperger CP, Schurgers LJ. The realm of vitamin K dependent proteins: shifting from coagulation toward calcification. Mol Nutr Food Res. 2014 Aug;58(8):1620-35. doi: 10.1002/mnfr.201300743[↩][↩]
- Sharma, B., & Albig, A. R. (2013). Matrix Gla protein reinforces angiogenic resolution. Microvascular research, 85, 24–33. https://doi.org/10.1016/j.mvr.2012.10.005[↩][↩]
- Sundaram K.S., Lev M. Warfarin administration reduces synthesis of sulfatides and other sphingolipids in mouse brain. J. Lipid Res. 1988;29:1475–1479. doi: 10.1016/S0022-2275(20)38426-1.[↩]
- Shafit-Zagardo, B., Gruber, R. C., & DuBois, J. C. (2018). The role of TAM family receptors and ligands in the nervous system: From development to pathobiology. Pharmacology & therapeutics, 188, 97–117. https://doi.org/10.1016/j.pharmthera.2018.03.002[↩]
- Simes, D. C., Viegas, C., Araújo, N., & Marreiros, C. (2020). Vitamin K as a Diet Supplement with Impact in Human Health: Current Evidence in Age-Related Diseases. Nutrients, 12(1), 138. https://doi.org/10.3390/nu12010138[↩]
- Ferland G. (2012). Vitamin K and the nervous system: an overview of its actions. Advances in nutrition (Bethesda, Md.), 3(2), 204–212. https://doi.org/10.3945/an.111.001784[↩]
- Weber P. Vitamin K and bone health. Nutrition. 2001 Oct;17(10):880-7. doi: 10.1016/s0899-9007(01)00709-2. Erratum in: Nutrition 2001 Nov-Dec;17(11-12):1024[↩][↩][↩][↩][↩][↩]
- Institute of Medicine. Dietary reference intakes for vitamin A, vitamin K, arsenic, boron, chromium, copper, iodine, iron, manganese, molybdenum, nickel, silicon, vanadium, and zinc. Washington, DC: National Academy Press; 2001.[↩][↩][↩][↩]
- Elder SJ, Haytowitz DB, Howe J, Peterson JW, Booth SL. Vitamin K contents of meat, dairy, and fast food in the U.S. Diet. J Agric Food Chem 2006;54:463-7. https://www.ncbi.nlm.nih.gov/pubmed/16417305?dopt=Abstract[↩][↩]
- Mott A, Bradley T, Wright K, Cockayne ES, Shearer MJ, Adamson J, Lanham-New SA, Torgerson DJ. Effect of vitamin K on bone mineral density and fractures in adults: an updated systematic review and meta-analysis of randomised controlled trials. Osteoporos Int. 2019 Aug;30(8):1543-1559. doi: 10.1007/s00198-019-04949-0. Epub 2019 May 10. Erratum in: Osteoporos Int. 2020 Nov;31(11):2269-2270.[↩][↩][↩]
- Oldenburg J, Bevans CG, Müller CR, Watzka M. Vitamin K epoxide reductase complex subunit 1 (VKORC1): the key protein of the vitamin K cycle. Antioxid Redox Signal. 2006 Mar-Apr;8(3-4):347-53. doi: 10.1089/ars.2006.8.347[↩]
- Vermeer C. Vitamin K: the effect on health beyond coagulation – an overview. Food Nutr Res 2012;56. doi: 10.3402/fnr.v56i0.5329[↩][↩][↩]
- Cheung AM, Tile L, Lee Y, Tomlinson G, Hawker G, Scher J, et al. Vitamin K supplementation in postmenopausal women with osteopenia (ECKO trial): a randomized controlled trial. PLoS Med 2008;5:e196.[↩][↩][↩]
- Cockayne S, Adamson J, Lanham-New S, Shearer MJ, Gilbody S, Torgerson DJ. Vitamin K and the prevention of fractures: systematic review and meta-analysis of randomized controlled trials. Arch Intern Med 2006;166:1256–61.[↩][↩][↩]
- Braam LA, Hoeks AP, Brouns F, Hamulyák K, Gerichhausen MJ, Vermeer C. Beneficial effects of vitamins D and K on the elastic properties of the vessel wall in postmenopausal women: a follow-up study. Thromb Haemost 2004;91:373–80.[↩][↩][↩]
- Villines TC, Hatzigeorgiou C, Feuerstein IM, O’Malley PG, Taylor AJ. Vitamin K1 intake and coronary calcification. Coron Artery Dis 2005;16:199–203.[↩][↩][↩]
- Habu D, Shiomi S, Tamori A, Takeda T, Tanaka T, Kubo S, et al. Role of vitamin K2 in the development of hepatocellular carcinoma in women with viral cirrhosis of the liver. J Am Med Assoc 2004;292:358–61.[↩][↩][↩]
- Hodges SJ, Pilkington MJ, Stamp TC. Depressed levels of circulating menaquinones in patients with osteoporotic fractures of the spine and femoral neck. Bone 1991;12:387–9.[↩][↩][↩]
- Orimo H, Shiraki M, Fujita T, Onomura T, Inoue T, Kushida K. Clinical evaluation of menatetrenone in the treatment of involutional osteoporosis-a double blind multicenter comparative study with 1α hydroxyvitamin D3. J Bone Miner Res 1992 (Suppl1);7:S122 (Abstract).[↩][↩][↩]
- Hara K, Akiyama Y, Ohkawa I, Tajima T. Effects of menatetrenone on prednisolone- induced bone loss in rats. Bone 1993;14:813–8.[↩][↩][↩]
- Akiyama Y, Hara K, Ohkawa I, Tajima T. Effects of menatetrenone on bone loss induced by ovariectomy in rats. Jpn J Pharmacol 1993;62:145–53.[↩][↩][↩]
- Koshihara Y, Hoshi K, Shiraki M. Vitamin K2 (menatetrenone) inhibits prostaglandin synthesis in cultured human osteoblast-like periosteal cells by inhibiting prostaglandin H synthase activity. Biochem Pharmacol 1993;46:1355–62.[↩][↩][↩]
- Akiyama Y, Hara K, Tajima T, Murota S, Morita I. Effect of vitamin K2 (menatetrenone) on osteoclast-like cell formation in mouse bone marrow cultures. Eur J Pharmacol 1994;263:181–5.[↩][↩][↩]
- Thomas DD, Krzuykowski KJ, Engelke JA, Groblewski GE. Exocrine pancreatic secretion of phospholipid, menaquinone-4, and caveolin-1 in vivo. Biochem Biophys Res Commun 2004;319:974–9.[↩][↩][↩]
- Fusaro M, Noale M, Viola V, Galli F, Tripepi G, Vajente N, et al. Vitamin K, vertebral fractures, vascular calcifications and mortality: VItamin K Italian (VIKI) dialysis study. J Bone Mineral Res 2012;27:2271–8.[↩][↩][↩][↩][↩][↩]
- Danziger J. Vitamin K-dependent proteins, warfarin, and vascular calcification. Clin J Am Soc Nephrol 2008;3:1504–10.[↩][↩][↩]
- Tufano A, Coppola A, Contaldi P, Franchini M, Minno GD. Oral anticoagulant drugs and the risk of osteoporosis: new anticoagulants better than old? Semin Thromb Hemost 2015;41:382–8.[↩][↩][↩]
- Fusaro M, Dalle Carbonare L, Dusso A, Arcidiacono MV, Valenti MT, Aghi A, et al. Differential effects of dabigatran and warfarin on bone volume and structure in rats with normal renal function. PLoS One 2015;10:e0133847.[↩][↩][↩]
- Shea MK, Booth SL, Massaro JM, Jacques PF, D’Agostino RB Sr, Dawson-Hughes B, et al. Vitamin K and vitamin D status: associations with inflammatory markers in the Framingham Offspring Study. Am J Epidemiol 2008;167:313–20.[↩][↩][↩]
- Ohsaki Y, Shirakawa H, Hiwatashi K, Furokawa Y, Mizutani T, Komai M. Vitamin K suppresses lipopolysaccharide-induced inflammation in the rat. Biosci Biotechnol Biochem 2006;70:926–32.[↩][↩][↩]
- Gundberg, C. M., Lian, J. B., & Booth, S. L. (2012). Vitamin K-dependent carboxylation of osteocalcin: friend or foe?. Advances in nutrition (Bethesda, Md.), 3(2), 149–157. https://doi.org/10.3945/an.112.001834[↩][↩][↩][↩]
- Hauschka PV, Lian JB, Cole DE, Gundberg CM. Osteocalcin and matrix Gla protein: vitamin K-dependent proteins in bone. Physiol Rev. 1989 Jul;69(3):990-1047. doi: 10.1152/physrev.1989.69.3.990[↩]
- Vermeer C, Theuwissen E. Vitamin K, osteoporosis and degenerative diseases of ageing. Menopause Int. 2011 Mar;17(1):19-23. doi: 10.1258/mi.2011.011006[↩]
- EFSA Panel on Dietetic Products Nutrition, and Allergies (NDA). Scientific opinion on the substantiation of health claims related to vitamin K and maintenance of bones (ID 123, 127, 128 and 2879), blood coagulation (ID 124 and 126), and function of the heart and blood vessels (ID 124, 125 and 2880) pursuant to Article 13(1) of Regulation (EC) No 1924/2006 on request from the European Commission. EFSA J. 2009;7:1228.[↩]
- Schurgers LJ, Teunissen KJ, Hamulyák K, Knapen MH, Vik H, Vermeer C. Vitamin K-containing dietary supplements: comparison of synthetic vitamin K1 and natto-derived menaquinone-7. Blood. 2007 Apr 15;109(8):3279-83. doi: 10.1182/blood-2006-08-040709[↩]
- Cockayne S, Adamson J, Lanham-New S, Shearer MJ, Gilbody S, Torgerson DJ. Vitamin K and the prevention of fractures: systematic review and meta-analysis of randomized controlled trials. Arch Intern Med. 2006 Jun 26;166(12):1256-61. doi: 10.1001/archinte.166.12.1256. Erratum in: JAMA Intern Med. 2018 Jun 1;178(6):875-876.[↩]
- Fang Y, Hu C, Tao X, Wan Y, Tao F. Effect of vitamin K on bone mineral density: a meta-analysis of randomized controlled trials. J Bone Miner Metab. 2012 Jan;30(1):60-8. doi: 10.1007/s00774-011-0287-3[↩]
- Forli L, Bollerslev J, Simonsen S, Isaksen GA, Kvamsdal KE, Godang K, Gadeholt G, Pripp AH, Bjortuft O. Dietary vitamin K2 supplement improves bone status after lung and heart transplantation. Transplantation. 2010 Feb 27;89(4):458-64. doi: 10.1097/TP.0b013e3181c46b69[↩]
- Emaus N, Gjesdal CG, Almås B, Christensen M, Grimsgaard AS, Berntsen GK, Salomonsen L, Fønnebø V. Vitamin K2 supplementation does not influence bone loss in early menopausal women: a randomised double-blind placebo-controlled trial. Osteoporos Int. 2010 Oct;21(10):1731-40. doi: 10.1007/s00198-009-1126-4[↩]
- Inoue T, Fujita T, Kishimoto H, Makino T, Nakamura T, Nakamura T, Sato T, Yamazaki K. Randomized controlled study on the prevention of osteoporotic fractures (OF study): a phase IV clinical study of 15-mg menatetrenone capsules. J Bone Miner Metab. 2009;27(1):66-75. doi: 10.1007/s00774-008-0008-8[↩]
- Kanellakis S, Moschonis G, Tenta R, Schaafsma A, van den Heuvel EG, Papaioannou N, Lyritis G, Manios Y. Changes in parameters of bone metabolism in postmenopausal women following a 12-month intervention period using dairy products enriched with calcium, vitamin D, and phylloquinone (vitamin K1) or menaquinone-7 (vitamin K (2[↩]
- Knapen MH, Drummen NE, Smit E, Vermeer C, Theuwissen E. Three-year low-dose menaquinone-7 supplementation helps decrease bone loss in healthy postmenopausal women. Osteoporos Int. 2013 Sep;24(9):2499-507. doi: 10.1007/s00198-013-2325-6[↩]
- Apalset EM, Gjesdal CG, Eide GE, Tell GS. Intake of vitamin K1 and K2 and risk of hip fractures: The Hordaland Health Study. Bone. 2011 Nov;49(5):990-5. doi: 10.1016/j.bone.2011.07.035[↩]
- Theuwissen E, Teunissen KJ, Spronk HM, Hamulyák K, Ten Cate H, Shearer MJ, Vermeer C, Schurgers LJ. Effect of low-dose supplements of menaquinone-7 (vitamin K2 ) on the stability of oral anticoagulant treatment: dose-response relationship in healthy volunteers. J Thromb Haemost. 2013 Jun;11(6):1085-92. doi: 10.1111/jth.12203[↩]
- Wei, G., Wang, M., Hyslop, T., Wang, Z., & Carr, B. I. (2010). Vitamin K enhancement of sorafenib-mediated HCC cell growth inhibition in vitro and in vivo. International journal of cancer, 127(12), 2949–2958. https://doi.org/10.1002/ijc.25498[↩]
- Tokita H, Tsuchida A, Miyazawa K, Ohyashiki K, Katayanagi S, Sudo H, Enomoto M, Takagi Y, Aoki T. Vitamin K2-induced antitumor effects via cell-cycle arrest and apoptosis in gastric cancer cell lines. Int J Mol Med. 2006 Feb;17(2):235-43.[↩]
- Yoshida T, Miyazawa K, Kasuga I, Yokoyama T, Minemura K, Ustumi K, Aoshima M, Ohyashiki K. Apoptosis induction of vitamin K2 in lung carcinoma cell lines: the possibility of vitamin K2 therapy for lung cancer. Int J Oncol. 2003 Sep;23(3):627-32.[↩]
- Karasawa S, Azuma M, Kasama T, Sakamoto S, Kabe Y, Imai T, Yamaguchi Y, Miyazawa K, Handa H. Vitamin K2 covalently binds to Bak and induces Bak-mediated apoptosis. Mol Pharmacol. 2013 Mar;83(3):613-20. doi: 10.1124/mol.112.082602[↩]
- Habu D, Shiomi S, Tamori A, Takeda T, Tanaka T, Kubo S, Nishiguchi S. Role of vitamin K2 in the development of hepatocellular carcinoma in women with viral cirrhosis of the liver. JAMA. 2004 Jul 21;292(3):358-61. doi: 10.1001/jama.292.3.358[↩]
- Yoshida H, Shiratori Y, Kudo M, Shiina S, Mizuta T, Kojiro M, Yamamoto K, Koike Y, Saito K, Koyanagi N, Kawabe T, Kawazoe S, Kobashi H, Kasugai H, Osaki Y, Araki Y, Izumi N, Oka H, Tsuji K, Toyota J, Seki T, Osawa T, Masaki N, Ichinose M, Seike M, Ishikawa A, Ueno Y, Tagawa K, Kuromatsu R, Sakisaka S, Ikeda H, Kuroda H, Kokuryu H, Yamashita T, Sakaida I, Katamoto T, Kikuchi K, Nomoto M, Omata M. Effect of vitamin K2 on the recurrence of hepatocellular carcinoma. Hepatology. 2011 Aug;54(2):532-40. doi: 10.1002/hep.24430[↩]
- Verso M, Agnelli G. New and old anticoagulants in cancer. Thromb Res. 2012 Apr;129 Suppl 1:S101-5. doi: 10.1016/S0049-3848(12)70027-0[↩]
- Nimptsch K, Rohrmann S, Kaaks R, Linseisen J. Dietary vitamin K intake in relation to cancer incidence and mortality: results from the Heidelberg cohort of the European Prospective Investigation into Cancer and Nutrition (EPIC-Heidelberg). Am J Clin Nutr. 2010 May;91(5):1348-58. doi: 10.3945/ajcn.2009.28691[↩]
- Karwowski, W., Naumnik, B., Szczepański, M., & Myśliwiec, M. (2012). The mechanism of vascular calcification – a systematic review. Medical science monitor : international medical journal of experimental and clinical research, 18(1), RA1–RA11. https://doi.org/10.12659/msm.882181[↩]
- Chatrou ML, Winckers K, Hackeng TM, Reutelingsperger CP, Schurgers LJ. Vascular calcification: the price to pay for anticoagulation therapy with vitamin K-antagonists. Blood Rev. 2012 Jul;26(4):155-66. doi: 10.1016/j.blre.2012.03.002[↩]
- Theuwissen, E., Smit, E., & Vermeer, C. (2012). The role of vitamin K in soft-tissue calcification. Advances in nutrition (Bethesda, Md.), 3(2), 166–173. https://doi.org/10.3945/an.111.001628[↩]
- Shea, M. K., O’Donnell, C. J., Hoffmann, U., Dallal, G. E., Dawson-Hughes, B., Ordovas, J. M., Price, P. A., Williamson, M. K., & Booth, S. L. (2009). Vitamin K supplementation and progression of coronary artery calcium in older men and women. The American journal of clinical nutrition, 89(6), 1799–1807. https://doi.org/10.3945/ajcn.2008.27338[↩]
- Shanahan CM, Proudfoot D, Farzaneh-Far A, Weissberg PL. The role of Gla proteins in vascular calcification. Crit Rev Eukaryot Gene Expr. 1998;8(3-4):357-75. doi: 10.1615/critreveukargeneexpr.v8.i3-4.60[↩]
- Braam L, McKeown N, Jacques P, Lichtenstein A, Vermeer C, Wilson P, Booth S. Dietary phylloquinone intake as a potential marker for a heart-healthy dietary pattern in the Framingham Offspring cohort. J Am Diet Assoc. 2004 Sep;104(9):1410-4. doi: 10.1016/j.jada.2004.06.021[↩]
- Geleijnse JM, Vermeer C, Grobbee DE, Schurgers LJ, Knapen MH, van der Meer IM, Hofman A, Witteman JC. Dietary intake of menaquinone is associated with a reduced risk of coronary heart disease: the Rotterdam Study. J Nutr. 2004 Nov;134(11):3100-5. doi: 10.1093/jn/134.11.3100[↩][↩][↩]
- Beulens JW, Bots ML, Atsma F, Bartelink ML, Prokop M, Geleijnse JM, Witteman JC, Grobbee DE, van der Schouw YT. High dietary menaquinone intake is associated with reduced coronary calcification. Atherosclerosis. 2009 Apr;203(2):489-93. doi: 10.1016/j.atherosclerosis.2008.07.010[↩][↩]
- Sato, T., Schurgers, L. J., & Uenishi, K. (2012). Comparison of menaquinone-4 and menaquinone-7 bioavailability in healthy women. Nutrition journal, 11, 93. https://doi.org/10.1186/1475-2891-11-93[↩][↩]
- Schurgers LJ, Vermeer C. Determination of phylloquinone and menaquinones in food. Effect of food matrix on circulating vitamin K concentrations. Haemostasis. 2000 Nov-Dec;30(6):298-307. doi: 10.1159/000054147[↩][↩][↩][↩]
- Erkkilä AT, Booth SL. Vitamin K intake and atherosclerosis. Curr Opin Lipidol. 2008 Feb;19(1):39-42. doi: 10.1097/MOL.0b013e3282f1c57f[↩]
- German JB, Dillard CJ. Composition, structure and absorption of milk lipids: a source of energy, fat-soluble nutrients and bioactive molecules. Crit Rev Food Sci Nutr. 2006;46(1):57-92. doi: 10.1080/10408690590957098[↩]
- NattoPharma.com[Internet] MENAQ7®: 3 year human study with vitamin K2 demonstrates that MenaQ7® significantly improves bone strength, and prevents cardiovascular aging.[↩]
- Trumbo P, Yates AA, Schlicker S, Poos M. Dietary reference intakes: vitamin A, vitamin K, arsenic, boron, chromium, copper, iodine, iron, manganese, molybdenum, nickel, silicon, vanadium, and zinc. J Am Diet Assoc. 2001 Mar;101(3):294-301. doi: 10.1016/S0002-8223(01)00078-5[↩][↩][↩]
- Dietary reference values for food energy and nutrients for the United Kingdom. Report on health and social subjects no. 41. London: HMSO; 1991.[↩]
- Kamao M, Suhara Y, Tsugawa N, Uwano M, Yamaguchi N, Uenishi K, Ishida H, Sasaki S, Okano T. Vitamin K content of foods and dietary vitamin K intake in Japanese young women. J Nutr Sci Vitaminol (Tokyo). 2007 Dec;53(6):464-70. doi: 10.3177/jnsv.53.464[↩][↩][↩][↩]
- Paiva SA, Sepe TE, Booth SL, Camilo ME, O’Brien ME, Davidson KW, Sadowski JA, Russell RM. Interaction between vitamin K nutriture and bacterial overgrowth in hypochlorhydria induced by omeprazole. Am J Clin Nutr. 1998 Sep;68(3):699-704. doi: 10.1093/ajcn/68.3.699[↩]
- Schurgers L, Geleijnse J, Grobbee D, Pols H, Hofman A, Witteman J, et al. Nutritional intake of vitamins K1 (phylloquinone) and K2 (menaquinone) in the Netherlands. J Nutr Environ Med. 1999;9:115–22.[↩]
- Vitamin K. https://ods.od.nih.gov/factsheets/VitaminK-Consumer[↩][↩]
- Vermeer, C., Raes, J., van ‘t Hoofd, C., Knapen, M., & Xanthoulea, S. (2018). Menaquinone Content of Cheese. Nutrients, 10(4), 446. https://doi.org/10.3390/nu10040446[↩]
- Hojo K, Watanabe R, Mori T, Taketomo N. Quantitative measurement of tetrahydromenaquinone-9 in cheese fermented by propionibacteria. J Dairy Sci. 2007 Sep;90(9):4078-83. doi: 10.3168/jds.2006-892[↩][↩]
- Kaneki M, Hodges SJ, Hosoi T, Fujiwara S, Lyons A, Crean SJ, Ishida N, Nakagawa M, Takechi M, Sano Y, Mizuno Y, Hoshino S, Miyao M, Inoue S, Horiki K, Shiraki M, Ouchi Y, Orimo H. Japanese fermented soybean food as the major determinant of the large geographic difference in circulating levels of vitamin K2: possible implications for hip-fracture risk. Nutrition. 2001 Apr;17(4):315-21. doi: 10.1016/s0899-9007(00)00554-2. Erratum in: Nutrition. 2006 Oct;22(10):1075.[↩]
- Morishita T, Tamura N, Makino T, Kudo S. Production of menaquinones by lactic acid bacteria. J Dairy Sci. 1999 Sep;82(9):1897-903. doi: 10.3168/jds.S0022-0302(99)75424-X[↩][↩]
- Manoury E, Jourdon K, Boyaval P, Fourcassié P. Quantitative measurement of vitamin K2 (menaquinones) in various fermented dairy products using a reliable high-performance liquid chromatography method. J Dairy Sci. 2013 Mar;96(3):1335-46. doi: 10.3168/jds.2012-5494[↩][↩][↩]
- Furuichi K, Hojo K, Katakura Y, Ninomiya K, Shioya S. Aerobic culture of Propionibacterium freudenreichii ET-3 can increase production ratio of 1,4-dihydroxy-2-naphthoic acid to menaquinone. J Biosci Bioeng. 2006 Jun;101(6):464-70. doi: 10.1263/jbb.101.464[↩]
- Koivu-Tikkanen TJ, Ollilainen V, Piironen VI. Determination of phylloquinone and menaquinones in animal products with fluorescence detection after postcolumn reduction with metallic zinc. J Agric Food Chem. 2000 Dec;48(12):6325-31. doi: 10.1021/jf000638u[↩]
- Britt, R. B., & Brown, J. N. (2018). Characterizing the Severe Reactions of Parenteral Vitamin K1. Clinical and applied thrombosis/hemostasis : official journal of the International Academy of Clinical and Applied Thrombosis/Hemostasis, 24(1), 5–12. https://doi.org/10.1177/1076029616674825[↩][↩]
- Asakura H, Myou S, Ontachi Y, Mizutani T, Kato M, Saito M, Morishita E, Yamazaki M, Nakao S. Vitamin K administration to elderly patients with osteoporosis induces no hemostatic activation, even in those with suspected vitamin K deficiency. Osteoporos Int. 2001 Dec;12(12):996-1000. doi: 10.1007/s001980170007[↩][↩]
- Ushiroyama T, Ikeda A, Ueki M. Effect of continuous combined therapy with vitamin K(2) and vitamin D(3) on bone mineral density and coagulofibrinolysis function in postmenopausal women. Maturitas. 2002 Mar 25;41(3):211-21. doi: 10.1016/s0378-5122(01)00275-4[↩][↩]
- Burke CW. Vitamin K deficiency bleeding: overview and considerations. J Pediatr Health Care. May-June 2013. 27:215-21.[↩][↩][↩]
- Fechtner RD, Minckler D, Weinreb RN, Frangei G, Jampol LM. Complications of glaucoma surgery. Ocular decompression retinopathy. Arch Ophthalmol. 1992 Jul;110(7):965-8. doi: 10.1001/archopht.1992.01080190071032[↩]
- Schurgers LJ. Vitamin K: key vitamin in controlling vascular calcification in chronic kidney disease. Kidney Int. 2013 May;83(5):782-4. doi: 10.1038/ki.2013.26[↩]
- Fusaro M, Crepaldi G, Maggi S, Galli F, D’Angelo A, Calò L, Giannini S, Miozzo D, Gallieni M. Vitamin K, bone fractures, and vascular calcifications in chronic kidney disease: an important but poorly studied relationship. J Endocrinol Invest. 2011 Apr;34(4):317-23. doi: 10.1007/BF03347093[↩]
- Schurgers LJ. Vitamin K: key vitamin in controlling vascular calcification in chronic kidney disease. Kidney Int2013;83:782-4. https://www.ncbi.nlm.nih.gov/pubmed/23633049?dopt=Abstract[↩][↩][↩]
- Takada T, Yamanashi Y, Konishi K, Yamamoto T, Toyoda Y, Masuo Y, Yamamoto H, Suzuki H. NPC1L1 is a key regulator of intestinal vitamin K absorption and a modulator of warfarin therapy. Sci Transl Med. 2015 Feb 18;7(275):275ra23. doi: 10.1126/scitranslmed.3010329[↩]
- Tie JK, Stafford DW. Functional Study of the Vitamin K Cycle Enzymes in Live Cells. Methods Enzymol. 2017;584:349-394. doi: 10.1016/bs.mie.2016.10.015[↩]
- Rishavy MA, Hallgren KW, Wilson LA, Usubalieva A, Runge KW, Berkner KL. The vitamin K oxidoreductase is a multimer that efficiently reduces vitamin K epoxide to hydroquinone to allow vitamin K-dependent protein carboxylation. J Biol Chem. 2013 Nov 1;288(44):31556-66. doi: 10.1074/jbc.M113.497297[↩]
- Tie JK, Jin DY, Straight DL, Stafford DW. Functional study of the vitamin K cycle in mammalian cells. Blood. 2011 Mar 10;117(10):2967-74. doi: 10.1182/blood-2010-08-304303[↩]
- Lacombe, J., Rishavy, M. A., Berkner, K. L., & Ferron, M. (2018). VKOR paralog VKORC1L1 supports vitamin K-dependent protein carboxylation in vivo. JCI insight, 3(1), e96501. https://doi.org/10.1172/jci.insight.96501[↩]
- Wajih, N.; Owen, J.; Wallin, R. Enhanced functional recombinant factor VII production by HEK 293 cells stably transfected with VKORC1 where the gamma-carboxylase inhibitor calumenin is stably suppressed by shRNA transfection. Thromb. Res. 2008, 122, 405–410.[↩]
- Violi F, Lip GY, Pignatelli P, Pastori D. Interaction Between Dietary Vitamin K Intake and Anticoagulation by Vitamin K Antagonists: Is It Really True?: A Systematic Review. Medicine (Baltimore). 2016 Mar;95(10):e2895. doi: 10.1097/MD.0000000000002895[↩][↩]
- Thijssen, H.H.; Vervoort, L.M.; Schurgers, L.J.; Shearer, M.J. Menadione is a metabolite of oral vitamin K. Br. J. Nutr. 2006, 95, 260–266.[↩][↩]
- Alisi, L., Cao, R., De Angelis, C., Cafolla, A., Caramia, F., Cartocci, G., Librando, A., & Fiorelli, M. (2019). The Relationships Between Vitamin K and Cognition: A Review of Current Evidence. Frontiers in neurology, 10, 239. https://doi.org/10.3389/fneur.2019.00239[↩]
- Vermeer, C.; van’t Hoofd, C.; Knapen, M.H.J.; Xanthoulea, S. Synthesis of 2-methyl-1,4-naphthoquinones with higher gamma-glutamyl carboxylase activity than MK-4 both in vitro and in vivo. Bioorg. Med. Chem. Lett. 2017, 27, 208–211.[↩]
- Fujii S, Shimizu A, Takeda N, Oguchi K, Katsurai T, Shirakawa H, Komai M, Kagechika H. Systematic synthesis and anti-inflammatory activity of ω-carboxylated menaquinone derivatives–Investigations on identified and putative vitamin K₂ metabolites. Bioorg Med Chem. 2015 May 15;23(10):2344-52. doi: 10.1016/j.bmc.2015.03.070[↩]
- Traber MG. Vitamin E and K interactions: a 50-year-old problem. Nutr Rev 2008;66:624–9.[↩]
- Tabb MM, Sun A, Zhou C, Grün F, Errandi J, Romero K, Pham H, Inoue S, Mallick S, Lin M, Forman BM, Blumberg B. Vitamin K2 regulation of bone homeostasis is mediated by the steroid and xenobiotic receptor SXR. J Biol Chem. 2003 Nov 7;278(45):43919-27. doi: 10.1074/jbc.M303136200[↩][↩]
- Xie, W.; Barwick, J.L.; Downes, M.; Blumberg, B.; Simon, C.M.; Nelson, M.C.; Neuschwander-Tetri, B.A.; Brunt, E.M.; Guzelian, P.S.; Evans, R.M. Humanized xenobiotic response in mice expressing nuclear receptor SXR. Nature 2000, 406, 435–439.[↩][↩]
- Ichikawa T, Horie-Inoue K, Ikeda K, Blumberg B, Inoue S. Steroid and xenobiotic receptor SXR mediates vitamin K2-activated transcription of extracellular matrix-related genes and collagen accumulation in osteoblastic cells. J Biol Chem. 2006 Jun 23;281(25):16927-16934. doi: 10.1074/jbc.M600896200[↩]
- Azuma K, Urano T, Ouchi Y, Inoue S. Vitamin K2 suppresses proliferation and motility of hepatocellular carcinoma cells by activating steroid and xenobiotic receptor. Endocr J. 2009;56(7):843-9. doi: 10.1507/endocrj.k09e-108[↩]
- Sada E, Abe Y, Ohba R, Tachikawa Y, Nagasawa E, Shiratsuchi M, Takayanagi R. Vitamin K2 modulates differentiation and apoptosis of both myeloid and erythroid lineages. Eur J Haematol. 2010 Dec;85(6):538-48. doi: 10.1111/j.1600-0609.2010.01530.x[↩]
- Ekins, S., Kortagere, S., Iyer, M., Reschly, E. J., Lill, M. A., Redinbo, M. R., & Krasowski, M. D. (2009). Challenges predicting ligand-receptor interactions of promiscuous proteins: the nuclear receptor PXR. PLoS computational biology, 5(12), e1000594. https://doi.org/10.1371/journal.pcbi.1000594[↩]
- Okano, T.; Shimomura, Y.; Yamane, M.; Suhara, Y.; Kamao, M.; Sugiura, M.; Nakagawa, K. Conversion of phylloquinone (Vitamin K1) into menaquinone-4 (Vitamin K2) in mice: Two possible routes for menaquinone-4 accumulation in cerebra of mice. J. Biol. Chem. 2008, 283, 11270–11279.[↩]
- Hirabayashi Y, Gotoh Y. Stage-dependent fate determination of neural precursor cells in mouse forebrain. Neurosci Res. 2005 Apr;51(4):331-6. doi: 10.1016/j.neures.2005.01.004[↩]
- Eriksson PS, Perfilieva E, Björk-Eriksson T, Alborn AM, Nordborg C, Peterson DA, Gage FH. Neurogenesis in the adult human hippocampus. Nat Med. 1998 Nov;4(11):1313-7. doi: 10.1038/3305[↩]
- Sakane, R.; Kimura, K.; Hirota, Y.; Ishizawa, M.; Takagi, Y.; Wada, A.; Kuwahara, S.; Makishima, M.; Suhara, Y. Synthesis of novel vitamin K derivatives with alkylated phenyl groups introduced at the ù-terminal side chain and evaluation of their neural differentiation activities. Bioorg. Med. Chem. Lett. 2017, 27, 4881–4884.[↩]
- Sadowski JA, Hood SJ, Dallal GE, Garry PJ. Phylloquinone in plasma from elderly and young adults: factors influencing its concentration. Am J Clin Nutr 1989;50:100-8. https://www.ncbi.nlm.nih.gov/pubmed/2750682?dopt=Abstract[↩][↩]
- Moshfegh A, Goldman, J., Cleveland, L. . What We Eat In America. NHANES 2001–2002: Usual Nutrient Intakes from Food Compared to Dietary Reference Intakes. U.S. Dept. of Agriculture, Agricultural Research Service. 2005.[↩][↩]
- Dietary reference intakes: vitamin A, vitamin K, arsenic, boron, chromium, copper, iodine, iron, manganese, molybdenum, nickel, silicon, vanadium, and zinc. Trumbo P, Yates AA, Schlicker S, Poos M. J Am Diet Assoc. 2001 Mar; 101(3):294-301. https://www.ncbi.nlm.nih.gov/pubmed/11269606/[↩]
- National Institutes of Health. Osteoporosis prevention, diagnosis, and therapy. NIH consensus statement 2000;17:1-45. https://www.ncbi.nlm.nih.gov/pubmed/11525451?dopt=Abstract[↩]
- Booth SL, Tucker KL, Chen H, et al. Dietary vitamin K intakes are associated with hip fracture but not with bone mineral density in elderly men and women. Am J Clin Nutr. 2000; 71:1201–8. https://www.ncbi.nlm.nih.gov/pubmed/10799384?dopt=Citation[↩]
- Booth SL, Broe KE, Gagnon DR, et al. Vitamin K intake and bone mineral density in women and men. Am J Clin Nutr. 2003; 77:512–6. https://www.ncbi.nlm.nih.gov/pubmed/12540415?dopt=Citation[↩]
- Feskanich D, Weber P, Willett WC, Rockett H, Booth SL, Colditz GA. Vitamin K intake and hip fractures in women: a prospective study. Am J Clin Nutr. 1999; 69:74–9. https://www.ncbi.nlm.nih.gov/pubmed/9925126?dopt=Citation[↩]
- Gundberg CM, Lian JB, Booth SL. Vitamin K-dependent carboxylation of osteocalcin: friend or foe? Adv Nutr 2012;3:149-57. https://www.ncbi.nlm.nih.gov/pubmed/22516722?dopt=Abstract[↩][↩]
- Yaegashi Y, Onoda T, Tanno K, Kuribayashi T, Sakata K, Orimo H. Association of hip fracture incidence and intake of calcium, magnesium, vitamin D, and vitamin K. Eur J Epidemiol 2008;23:219-25. https://www.ncbi.nlm.nih.gov/pubmed/18214692?dopt=Abstract[↩]
- Rejnmark L, Vestergaard P, Charles P, Hermann AP, Brot C, Eiken P, et al. No effect of vitamin K1 intake on bone mineral density and fracture risk in perimenopausal women. Osteoporos Int 2006;17:1122-32. https://www.ncbi.nlm.nih.gov/pubmed/16683180?dopt=Abstract[↩]
- Feskanich D, Weber P, Willett WC, Rockett H, Booth SL, Colditz GA. Vitamin K intake and hip fractures in women: a prospective study. Am J Clin Nutr 1999;69:74-9. https://www.ncbi.nlm.nih.gov/pubmed/9925126?dopt=Abstract[↩]
- Booth SL, Broe KE, Gagnon DR, Tucker KL, Hannan MT, McLean RR, et al. Vitamin K intake and bone mineral density in women and men. Am J Clin Nutr 2003;77:512-6. https://www.ncbi.nlm.nih.gov/pubmed/12540415?dopt=Abstract[↩]
- Booth SL, Tucker KL, Chen H, Hannan MT, Gagnon DR, Cupples LA, et al. Dietary vitamin K intakes are associated with hip fracture but not with bone mineral density in elderly men and women. Am J Clin Nutr 2000;71:1201-8. https://www.ncbi.nlm.nih.gov/pubmed/10799384?dopt=Abstract[↩]
- Chan R, Leung J, Woo J. No association between dietary vitamin K intake and fracture risk in chinese community-dwelling older men and women: a prospective study. Calcif Tissue Int 2012;90:396-403. https://www.ncbi.nlm.nih.gov/pubmed/22451220?dopt=Abstract[↩]
- Cockayne S, Adamson J, Lanham-New S, Shearer MJ, Gilbody S, Torgerson DJ. Vitamin K and the prevention of fractures: systematic review and meta-analysis of randomized controlled trials. Arch Intern Med 2006;166:1256-61. https://www.ncbi.nlm.nih.gov/pubmed/16801507?dopt=Abstract[↩]
- Knapen MH, Drummen NE, Smit E, Vermeer C, Theuwissen E. Three-year low-dose menaquinone-7 supplementation helps decrease bone loss in healthy postmenopausal women. Osteoporos Int 2013;24:2499-507. https://www.ncbi.nlm.nih.gov/pubmed/23525894?dopt=Abstract[↩]
- Booth SL, Dallal G, Shea MK, Gundberg C, Peterson JW, Dawson-Hughes B. Effect of vitamin K supplementation on bone loss in elderly men and women. J Clin Endocrinol Metab 2008;93:1217-23. https://www.ncbi.nlm.nih.gov/pubmed/18252784?dopt=Abstract[↩]
- Binkley N, Harke J, Krueger D, Engelke J, Vallarta-Ast N, Gemar D, et al. Vitamin K treatment reduces undercarboxylated osteocalcin but does not alter bone turnover, density, or geometry in healthy postmenopausal North American women. J Bone Miner Res 2009;24:983-91. https://www.ncbi.nlm.nih.gov/pubmed/19113922?dopt=Abstract[↩][↩]
- European Food Safety Authority. Scientific opinion on the substantiation of health claims related to vitamin K and maintenance of bone pursuant to Article 13(1) of Regulation (EC) No 1924/2006. The EFSA Journal 2009;7:1228.[↩]
- Jamal SA, Browner WS, Bauer DC, Cummings SR. Warfarin use and risk for osteoporosis in elderly women. Study of Osteoporotic Fractures Research Group. Ann Intern Med. 1998 May 15;128(10):829-32. doi: 10.7326/0003-4819-128-10-199805150-00006[↩]
- Caraballo PJ, Heit JA, Atkinson EJ, Silverstein MD, O’Fallon WM, Castro MR, Melton LJ 3rd. Long-term use of oral anticoagulants and the risk of fracture. Arch Intern Med. 1999 Aug 9-23;159(15):1750-6. doi: 10.1001/archinte.159.15.1750[↩]
- Gage BF, Birman-Deych E, Radford MJ, Nilasena DS, Binder EF. Risk of osteoporotic fracture in elderly patients taking warfarin: results from the National Registry of Atrial Fibrillation 2. Arch Intern Med. 2006 Jan 23;166(2):241-6. doi: 10.1001/archinte.166.2.241[↩]
- Caraballo PJ, Gabriel SE, Castro MR, Atkinson EJ, Melton LJ 3rd. Changes in bone density after exposure to oral anticoagulants: a meta-analysis. Osteoporos Int. 1999;9(5):441-8. doi: 10.1007/s001980050169[↩]
- Fusaro M, Crepaldi G, Maggi S, D’Angelo A, Calo L, Miozzo D, Fornasieri A, Gallieni M. Bleeding, vertebral fractures and vascular calcifications in patients treated with warfarin: hope for lower risks with alternative therapies. Curr Vasc Pharmacol. 2011 Nov;9(6):763-9. doi: 10.2174/157016111797484134[↩]
- Osteoarthritis (OA). https://www.cdc.gov/arthritis/basics/osteoarthritis.htm[↩]
- Harshman SG, Shea MK. The Role of Vitamin K in Chronic Aging Diseases: Inflammation, Cardiovascular Disease, and Osteoarthritis. Curr Nutr Rep. 2016 Jun;5(2):90-98. doi: 10.1007/s13668-016-0162-x[↩]
- Oka H, Akune T, Muraki S, En-yo Y, Yoshida M, Saika A, Sasaki S, Nakamura K, Kawaguchi H, Yoshimura N. Association of low dietary vitamin K intake with radiographic knee osteoarthritis in the Japanese elderly population: dietary survey in a population-based cohort of the ROAD study. J Orthop Sci. 2009 Nov;14(6):687-92. doi: 10.1007/s00776-009-1395-y[↩]
- Neogi T, Booth SL, Zhang YQ, Jacques PF, Terkeltaub R, Aliabadi P, Felson DT. Low vitamin K status is associated with osteoarthritis in the hand and knee. Arthritis Rheum. 2006 Apr;54(4):1255-61. doi: 10.1002/art.21735[↩]
- Misra D, Booth SL, Tolstykh I, Felson DT, Nevitt MC, Lewis CE, Torner J, Neogi T. Vitamin K deficiency is associated with incident knee osteoarthritis. Am J Med. 2013 Mar;126(3):243-8. doi: 10.1016/j.amjmed.2012.10.011[↩]
- Shea MK, Kritchevsky SB, Hsu FC, Nevitt M, Booth SL, Kwoh CK, McAlindon TE, Vermeer C, Drummen N, Harris TB, Womack C, Loeser RF; Health ABC Study. The association between vitamin K status and knee osteoarthritis features in older adults: the Health, Aging and Body Composition Study. Osteoarthritis Cartilage. 2015 Mar;23(3):370-8. doi: 10.1016/j.joca.2014.12.008[↩]
- Boer CG, Szilagyi I, Nguyen NL, Neogi T, Meulenbelt I, Ikram MA, Uitterlinden AG, Bierma-Zeinstra S, Stricker BH, van Meurs JB. Vitamin K antagonist anticoagulant usage is associated with increased incidence and progression of osteoarthritis. Ann Rheum Dis. 2021 May;80(5):598-604. doi: 10.1136/annrheumdis-2020-219483[↩]
- Neogi T, Felson DT, Sarno R, Booth SL. Vitamin K in hand osteoarthritis: results from a randomised clinical trial. Ann Rheum Dis. 2008 Nov;67(11):1570-3. doi: 10.1136/ard.2008.094771[↩]
- Demer LL, Tintut Y. Vascular calcification: pathobiology of a multifaceted disease. Circulation 2008;117:2938-48. https://www.ncbi.nlm.nih.gov/pubmed/18519861?dopt=Abstract[↩]
- Geleijnse JM, Vermeer C, Grobbee DE, Schurgers LJ, Knapen MH, van der Meer IM, et al. Dietary intake of menaquinone is associated with a reduced risk of coronary heart disease: the Rotterdam Study. J Nutr 2004;134:3100-5. https://www.ncbi.nlm.nih.gov/pubmed/15514282?dopt=Abstract[↩][↩]
- Beulens JW, Bots ML, Atsma F, Bartelink ML, Prokop M, Geleijnse JM, et al. High dietary menaquinone intake is associated with reduced coronary calcification. Atherosclerosis 2009;203:489-93. https://www.ncbi.nlm.nih.gov/pubmed/18722618?dopt=Abstract[↩]
- Shea MK, O’Donnell CJ, Hoffmann U, Dallal GE, Dawson-Hughes B, Ordovas JM, et al. Vitamin K supplementation and progression of coronary artery calcium in older men and women. Am J Clin Nutr 2009;89:1799-807. https://www.ncbi.nlm.nih.gov/pubmed/19386744?dopt=Abstract[↩][↩]
- Gallieni M, Fusaro M. Vitamin K and cardiovascular calcification in CKD: is patient supplementation on the horizon? Kidney Int 2014;86:232-4. https://www.ncbi.nlm.nih.gov/pubmed/25079019?dopt=Abstract[↩]
- CFR – Code of Federal Regulations Title 21. https://www.accessdata.fda.gov/scripts/cdrh/cfdocs/cfcfr/CFRSearch.cfm?fr=573.620[↩]
- Bolton-Smith C, Price RJ, Fenton ST, Harrington DJ, Shearer MJ. Compilation of a provisional UK database for the phylloquinone (vitamin K1) content of foods. Br J Nutr. 2000;83:389–99. https://www.ncbi.nlm.nih.gov/pubmed/10858697[↩]
- Pehrsson P, Haytowitz D, Holden J, Perry C, Beckler D. USDA’s National Food and Nutrient Analysis Program: food sampling. J Food Compos Anal. 2000;13:379–90.[↩]
- The USDA Food Composition Databases. https://ndb.nal.usda.gov/ndb/[↩]
- Booth SL. Vitamin K: food composition and dietary intakes. Food Nutr Res 2012;56. www.ncbi.nlm.nih.gov/pubmed/22489217?dopt=Abstract[↩][↩][↩]
- Food Labeling: Revision of the Nutrition and Supplement Facts Labels. https://www.federalregister.gov/documents/2016/05/27/2016-11867/food-labeling-revision-of-the-nutrition-and-supplement-facts-labels[↩]
- U.S. Department of Agriculture, Agricultural Research Service. USDA National Nutrient Database for Standard Reference, Release 28. Nutrient Data Laboratory Home Page, 2015. https://ndb.nal.usda.gov/ndb/[↩]
- National Institutes of Health. Dietary Supplement Label Database 2014. https://dsld.nlm.nih.gov/dsld/[↩][↩]
- Schurgers LJ, Teunissen KJ, Hamulyak K, Knapen MH, Vik H, Vermeer C. Vitamin K-containing dietary supplements: comparison of synthetic vitamin K1 and natto-derived menaquinone-7. Blood 2007;109:3279-83. https://www.ncbi.nlm.nih.gov/pubmed/17158229?dopt=Abstract[↩]
- Klebanoff MA, Read JS, Mills JL, Shiono PH. The risk of childhood cancer after neonatal exposure to vitamin K. N Engl J Med. 1993 Sep 23;329(13):905-8. doi: 10.1056/NEJM199309233291301[↩]
- Ekelund H, Finnström O, Gunnarskog J, Källén B, Larsson Y. Administration of vitamin K to newborn infants and childhood cancer. BMJ. 1993 Jul 10;307(6896):89-91. doi: 10.1136/bmj.307.6896.89[↩]
- Roman E, Fear NT, Ansell P, Bull D, Draper G, McKinney P, Michaelis J, Passmore SJ, von Kries R. Vitamin K and childhood cancer: analysis of individual patient data from six case-control studies. Br J Cancer. 2002 Jan 7;86(1):63-9. doi: 10.1038/sj.bjc.6600007[↩]
- American Academy of Pediatrics Committee on Fetus and Newborn. Controversies concerning vitamin K and the newborn. American Academy of Pediatrics Committee on Fetus and Newborn. Pediatrics. 2003 Jul;112(1 Pt 1):191-2.[↩][↩][↩]
- Majid A, Blackwell M, Broadbent RS, Barker DP, Al-Sallami HS, Edmonds L, Kerruish N, Wheeler BJ. Newborn Vitamin K Prophylaxis: A Historical Perspective to Understand Modern Barriers to Uptake. Hosp Pediatr. 2019 Jan;9(1):55-60. doi: 10.1542/hpeds.2018-0104[↩]
- Schulte R, Jordan LC, Morad A, Naftel RP, Wellons JC 3rd, Sidonio R. Rise in late onset vitamin K deficiency bleeding in young infants because of omission or refusal of prophylaxis at birth. Pediatr Neurol. 2014 Jun;50(6):564-8. doi: 10.1016/j.pediatrneurol.2014.02.013[↩]
- Costakos DT, Greer FR, Love LA, Dahlen LR, Suttie JW. Vitamin K prophylaxis for premature infants: 1 mg versus 0.5 mg. Am J Perinatol. 2003 Nov;20(8):485-90. doi: 10.1055/s-2003-45384[↩][↩]
- Kumar D, Greer FR, Super DM, Suttie JW, Moore JJ. Vitamin K status of premature infants: implications for current recommendations. Pediatrics. 2001 Nov;108(5):1117-22. doi: 10.1542/peds.108.5.1117[↩]
- Ardell S, Offringa M, Ovelman C, Soll R. Prophylactic vitamin K for the prevention of vitamin K deficiency bleeding in preterm neonates. Cochrane Database Syst Rev. 2018 Feb 5;2(2):CD008342. doi: 10.1002/14651858.CD008342.pub2[↩]
- Hendler SS, Rorvik DR, eds. PDR for Nutritional Supplements. Montvale: Medical Economics Company, Inc.; 2001.[↩]
- Drug-Nutrient Interaction Task Force, Clinical Center, National Institutes of Health. Important information to know when you are taking: warfarin (Coumadin) and vitamin K. 2012.[↩]
- Shearer MJ, Newman P. Recent trends in the metabolism and cell biology of vitamin K with special reference to vitamin K cycling and MK-4 biosynthesis. J Lipid Res. 2014 Mar;55(3):345-62. doi: 10.1194/jlr.R045559[↩]
- Chang CH, Wang YW, Yeh Liu PY, Kao Yang YH. A practical approach to minimize the interaction of dietary vitamin K with warfarin. J Clin Pharm Ther. 2014 Feb;39(1):56-60. doi: 10.1111/jcpt.12104[↩]
- Thorp JA, Gaston L, Caspers DR, Pal ML. Current concepts and controversies in the use of vitamin K. Drugs. 1995 Mar;49(3):376-87. doi: 10.2165/00003495-199549030-00005[↩]
- Reiffel JA. An important indirect drug interaction between dronedarone and warfarin that may be extrapolated to other drugs that can alter gastrointestinal function. Am Heart J. 2011 Feb;161(2):e5; author reply e7. doi: 10.1016/j.ahj.2010.11.013[↩]
- Shirolkar SC, Fiuzat M, Becker RC. Dronedarone and vitamin K antagonists: a review of drug-drug interactions. Am Heart J. 2010 Oct;160(4):577-82. doi: 10.1016/j.ahj.2010.07.008[↩]
- Vroonhof K, van Rijn HJ, van Hattum J. Vitamin K deficiency and bleeding after long-term use of cholestyramine. Neth J Med. 2003 Jan;61(1):19-21.[↩][↩]
- MacWalter RS, Fraser HW, Armstrong KM. Orlistat enhances warfarin effect. Ann Pharmacother. 2003 Apr;37(4):510-2. doi: 10.1345/aph.1C122[↩]
- McDuffie JR, Calis KA, Booth SL, Uwaifo GI, Yanovski JA. Effects of orlistat on fat-soluble vitamins in obese adolescents. Pharmacotherapy. 2002 Jul;22(7):814-22. doi: 10.1592/phco.22.11.814.33627[↩]
- Olson RE. Vitamin K. In: Shils ME, Olson JA, Shike M, Ross AC, eds. Modern Nutrition in Health and Disease. 9th ed. Baltimore: Williams & Wilkins; 1999:363-380.[↩][↩][↩][↩][↩]
- Traber MG. Vitamin E and K interactions–a 50-year-old problem. Nutr Rev. 2008 Nov;66(11):624-9. doi: 10.1111/j.1753-4887.2008.00123.x[↩]
- Booth SL, Golly I, Sacheck JM, Roubenoff R, Dallal GE, Hamada K, Blumberg JB. Effect of vitamin E supplementation on vitamin K status in adults with normal coagulation status. Am J Clin Nutr. 2004 Jul;80(1):143-8. doi: 10.1093/ajcn/80.1.143[↩]
- Pastori D, Carnevale R, Cangemi R, Saliola M, Nocella C, Bartimoccia S, Vicario T, Farcomeni A, Violi F, Pignatelli P. Vitamin E serum levels and bleeding risk in patients receiving oral anticoagulant therapy: a retrospective cohort study. J Am Heart Assoc. 2013 Oct 28;2(6):e000364. doi: 10.1161/JAHA.113.000364[↩]
- Merck Sharp & Dohme Corp., Merck Manual. Vitamin K. https://www.merckmanuals.com/professional/nutritional-disorders/vitamin-deficiency,-dependency,-and-toxicity/vitamin-k[↩][↩]
- Lee GR, Bithell TC, Forester J. Acquired coagulation disorders. Wintrobe’s Clinical Hematology. Baltimore, Md: Williams & Wilkins; 1993: 1473-80.[↩][↩]
- Jagannath VA, Fedorowicz Z, Thaker V, Chang AB. Vitamin K supplementation for cystic fibrosis. Cochrane Database Syst Rev. 2013 Apr 30;(4):CD008482. doi: 10.1002/14651858.CD008482.pub3. Update in: Cochrane Database Syst Rev. 2015;1:CD008482.[↩][↩][↩][↩]
- Nakajima S, Iijima H, Egawa S, Shinzaki S, Kondo J, Inoue T, Hayashi Y, Ying J, Mukai A, Akasaka T, Nishida T, Kanto T, Tsujii M, Hayashi N. Association of vitamin K deficiency with bone metabolism and clinical disease activity in inflammatory bowel disease. Nutrition. 2011 Oct;27(10):1023-8. doi: 10.1016/j.nut.2010.10.021[↩][↩]
- Nowak JK, Grzybowska-Chlebowczyk U, Landowski P, Szaflarska-Poplawska A, Klincewicz B, Adamczak D, Banasiewicz T, Plawski A, Walkowiak J. Prevalence and correlates of vitamin K deficiency in children with inflammatory bowel disease. Sci Rep. 2014 Apr 24;4:4768. doi: 10.1038/srep04768[↩][↩]
- Dong R, Wang N, Yang Y, Ma L, Du Q, Zhang W, Tran AH, Jung H, Soh A, Zheng Y, Zheng S. Review on Vitamin K Deficiency and its Biomarkers: Focus on the Novel Application of PIVKA-II in Clinical Practice. Clin Lab. 2018 Apr 1;64(4):413-424. doi: 10.7754/Clin.Lab.2017.171020[↩][↩][↩][↩][↩][↩]
- Mummah-Schendel LL, Suttie JW. Serum phylloquinone concentrations in a normal adult population. Am J Clin Nutr. 1986 Nov;44(5):686-9. doi: 10.1093/ajcn/44.5.686[↩]
- Riphagen IJ, Keyzer CA, Drummen NEA, de Borst MH, Beulens JWJ, Gansevoort RT, Geleijnse JM, Muskiet FAJ, Navis G, Visser ST, Vermeer C, Kema IP, Bakker SJL. Prevalence and Effects of Functional Vitamin K Insufficiency: The PREVEND Study. Nutrients. 2017 Dec 8;9(12):1334. doi: 10.3390/nu9121334[↩]
- Liebman HA, Furie BC, Tong MJ. Des-gamma-carboxy (abnormal) prothrombin as a serum marker of primary hepatocellular carcinoma. N Engl J Med. 1984 May 31. 310(22):1427-31.[↩][↩]
- Sokoll LJ, Sadowski JA. Comparison of biochemical indexes for assessing vitamin K nutritional status in a healthy adult population. Am J Clin Nutr. 1996 Apr;63(4):566-73. doi: 10.1093/ajcn/63.4.566[↩][↩]
- Suttie JW, Mummah-Schendel LL, Shah DV, Lyle BJ, Greger JL. Vitamin K deficiency from dietary vitamin K restriction in humans. Am J Clin Nutr. 1988 Mar;47(3):475-80. doi: 10.1093/ajcn/47.3.475[↩][↩]
- Mihatsch WA, Braegger C, Bronsky J, Campoy C, Domellöf M, Fewtrell M, Mis NF, Hojsak I, Hulst J, Indrio F, Lapillonne A, Mlgaard C, Embleton N, van Goudoever J; ESPGHAN Committee on Nutrition. Prevention of Vitamin K Deficiency Bleeding in Newborn Infants: A Position Paper by the ESPGHAN Committee on Nutrition. J Pediatr Gastroenterol Nutr. 2016 Jul;63(1):123-9. doi: 10.1097/MPG.0000000000001232[↩][↩][↩][↩][↩][↩]
- van Hasselt PM, de Koning TJ, Kvist N, et al. Prevention of vitamin K deficiency bleeding in breastfed infants: lessons from the Dutch and Danish biliary atresia registries. Pediatrics. 2008 Apr. 121(4):e857-63.[↩][↩]
- Brenner B., Tavori S., Zivelin A., Keller C.B., Suttie J.W., Tatarsky I., et al. Hereditary deficiency of all vitamin K-dependent procoagulants and anticoagulants. Br. J. Haematol. 1990;75:537–542. doi: 10.1111/j.1365-2141.1990.tb07795.x[↩]
- Boneh A., Bar-Ziv J. Hereditary deficiency of vitamin K-dependent coagulation factors with skeletal abnormalities. Am. J. Med. Genet. 1996;65:241–243. doi: 10.1002/(SICI)1096-8628(19961028)65:3<241::AID-AJMG13>3.0.CO;2-O[↩][↩]
- Brenner B. Hereditary deficiency of vitamin K-dependent coagulation factors. Thromb Haemost. 2000 Dec;84(6):935-6.[↩]
- Napolitano M, Mariani G, Lapecorella M. Hereditary combined deficiency of the vitamin K-dependent clotting factors. Orphanet J Rare Dis. 2010 Jul 14;5:21. doi: 10.1186/1750-1172-5-21[↩][↩][↩][↩][↩][↩][↩][↩]
- Ayyash M, Chitlur M, Oldenburg J, Shaman M. Suspected vitamin K-dependent coagulation factor deficiency in pregnancy: A case report. Case Rep Womens Health. 2022 Apr 27;34:e00416. doi: 10.1016/j.crwh.2022.e00416[↩]
- Kuo W.L., Stafford D.W., Cruces J., Gray J., Solera J. Chromosomal localization of the gamma-glutamyl carboxylase gene at 2p12. Genomics. 1995;25:746–748. doi: 10.1016/0888-7543(95)80024-g[↩]
- Fregin A., Rost S., Wolz W., Krebsova A., Muller C.R., Oldenburg J. Homozygosity mapping of a second gene locus for hereditary combined deficiency of vitamin K-dependent clotting factors to the centromeric region of chromosome 16. Blood. 2002;100:3229–3232. doi: 10.1182/blood-2002-03-0698[↩]
- McMillan C.W., Roberts H.R. Congenital combined deficiency of coagulation factors II, VII, IX and X. Report of a case. N. Engl. J. Med. 1966;274:1313–1315. doi: 10.1056/NEJM196606092742309[↩][↩]
- Owen C., Jr. In: A History of Blood Coagulation. Owen C.A., Nichols W.L., Bowie E.J.W., editors. Mayo Foundation for Medical Education and Research; Rochester, MN: 2001. Fibrinolysis and thrombolysis; pp. 87–96.[↩]
- Mingers A.M., Heimburger N., Zeitler P., Kreth H.W., Schuster V. Homozygous type I plasminogen deficiency. Semin. Thromb. Hemost. 1997;23:259–269. doi: 10.1055/s-2007-996099[↩]
- Weston B.W., Monahan P.E. Familial deficiency of vitamin K-dependent clotting factors. Haemophilia. 2008;14:1209–1213. doi: 10.1111/j.1365-2516.2008.01853.x[↩][↩]
- Goldsmith G.H., Jr., Pence R.E., Ratnoff O.D., Adelstein D.J., Furie B. Studies on a family with combined functional deficiencies of vitamin K-dependent coagulation factors. J. Clin. Invest. 1982;69:1253–1260. doi: 10.1172/jci110564[↩][↩]
- McMahon M.J., James A.H. Combined deficiency of factors II, VII, IX, and X (Borgschulte-Grigsby deficiency) in pregnancy. Obstet. Gynecol. 2001;97:808–809. doi: 10.1016/s0029-7844(00)01214-x[↩][↩][↩]
- Pauli RM, Lian JB, Mosher DF, Suttie JW. Association of congenital deficiency of multiple vitamin K-dependent coagulation factors and the phenotype of the warfarin embryopathy: clues to the mechanism of teratogenicity of coumarin derivatives. Am J Hum Genet. 1987 Oct;41(4):566-83. https://www.ncbi.nlm.nih.gov/pmc/articles/PMC1684308/pdf/ajhg00133-0054.pdf[↩][↩]
- Chung KS, Bezeaud A, Goldsmith JC, McMillan CW, Ménaché D, Roberts HR. Congenital deficiency of blood clotting factors II, VII, IX, and X. Blood. 1979 Apr;53(4):776-87. https://doi.org/10.1182/blood.V53.4.776.776[↩][↩]
- Vicente V, Maia R, Alberca I, Tamagnini GP, Lopez Borrasca A. Congenital deficiency of vitamin K-dependent coagulation factors and protein C. Thromb Haemost. 1984 Jul 29;51(3):343-6.[↩]
- Furie B, Furie BC. Molecular basis of vitamin K-dependent gamma-carboxylation. Blood. 1990 May 1;75(9):1753-62. https://doi.org/10.1182/blood.V75.9.1753.1753[↩]
- Leissinger C.A., Blatt P.M., Hoots W.K., Ewenstein B. Role of prothrombin complex concentrates in reversing warfarin anticoagulation: a review of the literature. Am. J. Hematol. 2008;83:137–143. doi: 10.1002/ajh.21046[↩]
- Booth SL, Al Rajabi A. Determinants of vitamin K status in humans. Vitam Horm. 2008. 78:1-22.[↩]
- Kristin M McCabe, Michael A. Adams, Rachel M. Holden. Vitamin K Status in Chronic Kidney Disease. Nutrition. November 2013. 5:4390-4398.[↩]
- Krasinski SD, Russell RM, Furie BC. The prevalence of vitamin K deficiency in chronic gastrointestinal disorders. Am J Clin Nutr. 1985 Mar. 41(3):639-43.[↩][↩]
- Jagannath VA, Fedorowicz Z, Thaker V, Chang AB. Vitamin K supplementation for cystic fibrosis. Cochrane Database Syst Rev. January 18, 2015. 1:1-33.[↩][↩]
- Shearer MJ. Vitamin K deficiency bleeding (VKDB) in early infancy. Blood Rev. 2008 Sep 18.[↩]
- Martin J. Shearer, Xueyan Fu, Sarah L. Booth. Vitamin K Nutrition, Metabolism, and Requirement: Current Concept and Future Research. Advances in Nutrition. 2012. 3:182-195.[↩][↩]
- Shearer MJ. Vitamin K deficiency bleeding (VKDB) in early infancy. Blood Rev. 2009 Mar;23(2):49-59. doi: 10.1016/j.blre.2008.06.001[↩][↩][↩][↩][↩]
- Ferland G. Vitamin K. In: ISLI, ed. Present Knowledge in Nutrition. 10th ed: John Wiley & Sons; 2012:230-247.[↩]
- Araki S, Shirahata A. Vitamin K Deficiency Bleeding in Infancy. Nutrients. 2020 Mar 16;12(3):780. doi: 10.3390/nu12030780[↩]
- Jullien S. Vitamin K prophylaxis in newborns. BMC Pediatr. 2021 Sep 8;21(Suppl 1):350. doi: 10.1186/s12887-021-02701-4[↩]
- Pichler E, Pichler L. The neonatal coagulation system and the vitamin K deficiency bleeding – a mini review. Wien Med Wochenschr. 2008;158(13-14):385-95. doi: 10.1007/s10354-008-0538-7[↩][↩]
- Jagannath VA, Fedorowicz Z, Thaker V, Chang AB. Vitamin K supplementation for cystic fibrosis. The Cochrane database of systematic reviews 2013;4:CD008482. https://www.ncbi.nlm.nih.gov/pubmed/25596954?dopt=Abstract[↩]
- Heber D, Greenway FL, Kaplan LM, Livingston E, Salvador J, Still C; Endocrine Society. Endocrine and nutritional management of the post-bariatric surgery patient: an Endocrine Society Clinical Practice Guideline. J Clin Endocrinol Metab. 2010 Nov;95(11):4823-43. doi: 10.1210/jc.2009-2128. Erratum in: J Clin Endocrinol Metab. 2021 May 13;106(6):e2459.[↩]
- Eden RE, Coviello JM. Vitamin K Deficiency. [Updated 2022 Jul 4]. In: StatPearls [Internet]. Treasure Island (FL): StatPearls Publishing; 2023 Jan-. Available from: https://www.ncbi.nlm.nih.gov/books/NBK536983[↩][↩][↩][↩][↩][↩][↩]
- Marchili MR, Santoro E, Marchesi A, Bianchi S, Rotondi Aufiero L, Villani A. Vitamin K deficiency: a case report and review of current guidelines. Ital J Pediatr. 2018 Mar 14;44(1):36. doi: 10.1186/s13052-018-0474-0[↩]
- Oldenburg J, von Brederlow B, Fregin A, Rost S, Wolz W, Eberl W, Eber S, Lenz E, Schwaab R, Brackmann HH, Effenberger W, Harbrecht U, Schurgers LJ, Vermeer C, Müller CR. Congenital deficiency of vitamin K dependent coagulation factors in two families presents as a genetic defect of the vitamin K-epoxide-reductase-complex. Thromb Haemost. 2000 Dec;84(6):937-41.[↩]
- Brenner B, Sánchez-Vega B, Wu SM, Lanir N, Stafford DW, Solera J. A missense mutation in gamma-glutamyl carboxylase gene causes combined deficiency of all vitamin K-dependent blood coagulation factors. Blood. 1998 Dec 15;92(12):4554-9. https://doi.org/10.1182/blood.V92.12.4554[↩]
- Spronk HM, Farah RA, Buchanan GR, Vermeer C, Soute BA. Novel mutation in the gamma-glutamyl carboxylase gene resulting in congenital combined deficiency of all vitamin K-dependent blood coagulation factors. Blood. 2000 Nov 15;96(10):3650-2. https://doi.org/10.1182/blood.V96.10.3650[↩]
- Brenner B., Kuperman A.A., Watzka M., Oldenburg J. Vitamin K-dependent coagulation factors deficiency. Semin. Thromb. Hemost. 2009;35:439–446. doi: 10.1055/s-0029-1225766[↩]
- Pechlaner C, Vogel W, Erhart R, Pümpel E, Kunz F. A new case of combined deficiency of vitamin K dependent coagulation factors. Thromb Haemost. 1992 Nov 10;68(5):617.[↩]
- Ghosh K., Shetty S., Mohanty D. Inherited deficiency of multiple vitamin K-dependent coagulation factors and coagulation inhibitors presenting as hemorrhagic diathesis, mental retardation, and growth retardation. Am. J. Hematol. 1996;52:67. doi: 10.1002/(SICI)1096-8652(199605)52:1<67::AID-AJH18>3.0.CO;2–4[↩]
- Takahashi D, Shirahata A, Itoh S, Takahashi Y, Nishiguchi T, Matsuda Y. Vitamin K prophylaxis and Late Vitamin K deficiency bleeding in infants: The 5th nation-wide survey in Japan. Pediatr Int. 2011 Apr 22.[↩]
- Nakajima S, Iijima H, Egawa S, Shinzaki S, Kondo J, Inoue T, et al. Association of vitamin K deficiency with bone metabolism and clinical disease activity in inflammatory bowel disease. Nutrition. 2011 Oct. 27(10):1023-8.[↩]
- Kuperman A., Brenner B. Clinical perspective of congenital vitamin K-dependent coagulation factor deficiency. J. Coagul. Disord. 2009;000(000[↩]
- Shearer MJ. Vitamin K deficiency bleeding (VKDB) in early infancy. Blood Rev. 2008 Sep 18[↩]
- Baglin TP, Rose PE. Guidelines on oral anticoagulation: third edition. Br J Haematol. 1998;101:374–387. doi: 10.1046/j.1365-2141.1998.00715.x[↩][↩]
- Leissinger CA, Blatt PM, Hoors WK, Ewenstein B. Role of prothrombin complex concentrates in reversing warfarin anticoagulation: a review of the literature. Am J Hematol. 2008;83:137–143. doi: 10.1002/ajh.21046[↩]
- Sathe MN, Patel AS. Update in pediatrics: focus on fat-soluble vitamins. Nutr Clin Pract. 2010 Aug;25(4):340-6. doi: 10.1177/0884533610374198[↩]
- Frequently Asked Questions (FAQ’s): Vitamin K and the Vitamin K Shot Given at Birth. https://www.cdc.gov/ncbddd/vitamink/faqs.html[↩]